Metrological characterization of porosity graded β-Ti21S triply periodic minimal surface cellular structure manufactured by laser powder bed fusion
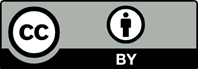
The design of a functionally graded porous structure (FGPS) for use in prosthetic devices is crucial for meeting both mechanical and biological requirements. One of the most commonly used cellular structures in FGPS is the triply periodic minimal surface (TPMS) structure due to its ability to be defined by implicit equations, which allows for smooth transitions between layers. This study evaluates the feasibility of using a novel β-Ti21S alloy to fabricate TPMS-based FGPS. This beta titanium alloy exhibits low elastic modulus (53 GPa) and good mechanical properties in as-built condition. Two TPMS FGPSs with relative density gradients of 0.17, 0.34, 0.50, 0.66, and 0.83 and unit cell sizes of 2.5 mm and 4 mm were designed and fabricated using laser powder bed fusion (LPBF). The as-manufactured structures were analyzed using scanning electron microscopy (SEM) and X-ray micro-computed tomography (µ-CT), and the results were compared to the design. The analysis revealed that the pore size and ligament thickness were undersized by less than 5%. Compression tests showed that the stabilized elastic modulus was 4.1 GPa for the TPMS with a 2.5 mm unit cell size and 10.7 GPa for the TPMS with a 4 mm unit cell size. A finite element simulation was performed to predict the specimen’s elastic properties, and a lumped model based on lattice homogenized properties was proposed and its limitations were explored.
1. Sam Froes FH, Qian M, Niinomi M, 2019, Titanium for Consumer Applications: Real World Use of Titanium, 1–349. https://doi.org/10.1016/C2017-0-03513-9
2. Balakrishnan P, Sreekala MS, Thomas S, 2018, Fundamental
Biomaterials: Metals, 1–450. https://doi.org/10.1016/C2016-0-03502-7
3. Standard Specification for Wrought Titanium-6Aluminum- 4Vanadium ELI (Extra Low Interstitial) Alloy for Surgical Implant Applications (UNS R56401). https://www.astm.org/f0136-13r21e01.html (accessed December 7, 2022).
4. Geetha M, Singh AK, Asokamani R, et al., 2009, Ti based biomaterials, the ultimate choice for orthopaedic implants: A review. Prog Mater Sci, 54:397–425. https://doi.org/10.1016/J.PMATSCI.2008.06.004
5. Rao S, Ushida T, Tateishi T, et al., 1996, Effect of Ti, Al, and V ions on the relative growth rate of fibroblasts (L929) and osteoblasts (MC3T3-E1) cells. Biomed Mater Eng, 6:79–86. https://doi.org/10.3233/BME-1996-6202
6. Wang M, Lin F, Zhang X, et al., 2022, Combination of alpinia oxyphylla fructus and schisandra chinensis fructus ameliorates aluminum-induced Alzheimer’s disease via reducing BACE1 expression. J Chem Neuroanat, 126: 102180. https://doi.org/10.1016/J.JCHEMNEU.2022.102180
7. Abdel-Hady Gepreel M, Niinomi M, 2013, Biocompatibility of Ti-alloys for long-term implantation. J Mech Behav Biomed Mater, 20:407–415. https://doi.org/10.1016/J.JMBBM.2012.11.014
8. Kumar A, Nune KC, Misra RDK, 2016, Biological functionality and mechanistic contribution of extracellular matrix-ornamented three dimensional Ti-6Al-4V mesh scaffolds. J Biomed Mater Res Part A, 104:2751–2763. https://doi.org/10.1002/JBM.A.35809.
9. ASTM F2066-18 - Standard Specification for Wrought Titanium-15 Molybdenum Alloy for Surgical Implant Applications (UNS R58150).
10. ASTM F1813-21 - Standard Specification for Wrought Titanium-12Molybdenum-6Zirconium-2Iron Alloy for Surgical Implant (UNS R58120).
11. ISO - ISO 5832-14:2019 - Implants for surgery—Metallic materials—Part 14: Wrought titanium 15-molybdenum 5-zirconium 3-aluminium alloy.
12. Brunke F, Siemers C, Rösler J, 2020, Second-generation titanium alloys Ti-15Mo and Ti-13Nb-13Zr: A comparison of the mechanical properties for implant applications. MATEC Web Conf, 321:05006. https://doi.org/10.1051/matecconf/202032105006
13. Duan R, Li S, Cai B, et al., 2021, A high strength and low modulus metastable β Ti-12Mo-6Zr-2Fe alloy fabricated by laser powder bed fusion in-situ alloying. Addit Manuf, 37:101708. https://doi.org/10.1016/j.addma.2020.101708
14. Escobar Claros CA, Contri Campanelli L, Moreira Jorge A, et al., 2021, Corrosion behaviour of biomedical β-titanium alloys with the surface-modified by chemical etching and electrochemical methods. Corros Sci, 188:109544. https://doi.org/10.1016/J.CORSCI.2021.109544
15. Materials Properties Handbook: Titanium Alloys, ASM International. https://www.asminternational.org/materials-resources/results//journal_content/56/10192/06005G/PUBLICATION (accessed 13 July 2022).
16. Macias-Sifuentes MA, Xu C, Sanchez-Mata O, et al., 2021, Microstructure and mechanical properties of β-21S Ti alloy fabricated through laser powder bed fusion. Prog Addit Manuf, 6:417–430. https://doi.org/10.1007/s40964-021-00181-7
17. Pellizzari M, Jam A, Tschon M, et al., 2020, A 3D-printed ultra-low young’s modulus β-Ti alloy for biomedical applications. Materials (Basel), 13:1–16. https://doi.org/10.3390/ma13122792
18. Jam A, du Plessis A, Lora C, et al., 2022, Manufacturability of lattice structures fabricated by laser powder bed fusion: A novel biomedical application of the beta Ti-21S alloy. Addit Manuf, 50:102556. https://doi.org/10.1016/J.ADDMA.2021.102556
19. Gibson LJ, Ashby MF, Harley BA, Cellular Materials in Nature and Medicine, 309.
20. Ashby MF, 2006, The properties of foams and lattices. Philos Trans R Soc A Math Phys Eng Sci, 364:15–30. https://doi.org/10.1098/RSTA.2005.1678
21. Abate KM, Nazir A, Jeng JY, 2021, Design, optimization, and selective laser melting of vin tiles cellular structure-based hip implant. Int J Adv Manuf Technol, 112:2037–2050. https://doi.org/10.1007/S00170-020-06323-5
22. Stewart C, Akhavan B, Wise SG, et al., 2019, A review of biomimetic surface functionalization for bone-integrating orthopedic implants: Mechanisms, current approaches, and future directions. Prog Mater Sci, 106:100588. https://doi.org/10.1016/j.pmatsci.2019.100588
23. Bobbert FSL, Lietaert K., Eftekhari AA, et al., 2017, Additively manufactured metallic porous biomaterials based on minimal surfaces: A unique combination of topological, mechanical, and mass transport properties. Acta Biomater, 53:572–584. https://doi.org/10.1016/j.actbio.2017.02.024
24. Zhang J, Chen X, Sun Y, et al., 2022, Design of a biomimetic graded TPMS scaffold with quantitatively adjustable pore size. Mater Des, 218:110665. https://doi.org/10.1016/J.MATDES.2022.110665
25. Zheng Y, Han Q, Wang J, et al., 2020, Promotion of osseointegration between implant and bone interface by titanium alloy porous scaffolds prepared by 3D printing. ACS Biomater Sci Eng, 6:5181–5190. https://doi.org/10.1021/acsbiomaterials.0c00662
26. Al-Ketan O, Rowshan R, Abu Al-Rub RK, 2018, Topology-mechanical property relationship of 3D printed strut, skeletal, and sheet based periodic metallic cellular materials. Addit Manuf, 19:167–183. https://doi.org/10.1016/J.ADDMA.2017.12.006
27. Tang M, Pistorius PC, Beuth JL, 2017, Prediction of lack-of-fusion porosity for powder bed fusion. Addit Manuf, 14:39–48. https://doi.org/10.1016/J.ADDMA.2016.12.001
28. Alaña M, Cutolo A, Probst G, et al., 2020, Understanding elastic anisotropy in diamond based lattice structures produced by laser powder bed fusion: Effect of manufacturing deviations. Mater Des, 195:1–12. https://doi.org/10.1016/j.matdes.2020.108971
29. Emanuelli L, Jam A, Du Plessis A, et al., Manufacturability of functionally graded porous β-Ti21S auxetic architected biomaterials produced by laser powder bed fusion: Comparison between 2D and 3D metrological characterization. Int J Bioprint, 9(2): 0213.
30. Benedetti M, du Plessis A, Ritchie RO, et al., 2021, Architected cellular materials: A review on their mechanical properties towards fatigue-tolerant design and fabrication. Mater Sci Eng R Rep, 144:100606. https://doi.org/10.1016/j.mser.2021.100606
31. Al-Ketan O, Rowshan R, Abu Al-Rub RK, 2018, Topology-mechanical property relationship of 3D printed strut, skeletal, and sheet based periodic metallic cellular materials. Addit Manuf, 19:167–183. https://doi.org/10.1016/j.addma.2017.12.006
32. Yánez A, Cuadrado A, Martel O, et al., 2018, Gyroid porous titanium structures: A versatile solution to be used as scaffolds in bone defect reconstruction. Mater Des, 140:21–29. https://doi.org/10.1016/j.matdes.2017.11.050
33. Jin Y, Kong H, Zhou X, et al., 2020, Design and characterization of sheet-based gyroid porous structures with bioinspired functional gradients. Materials (Basel), 13: 108971. https://doi.org/10.3390/ma13173844
34. Yuan L, Ding S, Wen C, 2019, Additive manufacturing technology for porous metal implant applications and triple minimal surface structures: A review. Bioact Mater, 4:56–70. https://doi.org/10.1016/j.bioactmat.2018.12.003
35. Soro N, Saintier N, Merzeau J, et al., 2021, Quasi-static and fatigue properties of graded Ti–6Al–4V lattices produced by laser powder bed fusion (LPBF). Addit Manuf, 37:101653. https://doi.org/10.1016/j.addma.2020.101653
36. Yang L, Yan C, Han C, et al., 2018, Mechanical response of a triply periodic minimal surface cellular structures manufactured by selective laser melting. Int J Mech Sci, 148:149–157. https://doi.org/10.1016/j.ijmecsci.2018.08.039
37. Shi J, Zhu L, Li L, et al., 2018, A TPMS-based method for modeling porous scaffolds for bionic bone tissue engineering. Sci Rep, 8:7395. https://doi.org/10.1038/s41598-018-25750-9
38. Zadpoor AA, 2019, Mechanical performance of additively manufactured meta-biomaterials. Acta Biomater, 85:41–59. https://doi.org/10.1016/j.actbio.2018.12.038
39. Kapfer SC, Hyde ST, Mecke K, et al., 2011, Minimal surface scaffold designs for tissue engineering. Biomaterials, 32:6875–6882. https://doi.org/10.1016/J.BIOMATERIALS.2011.06.012
40. Zhao S, Li SJ, Wang SG, et al., 2018, Compressive and fatigue behavior of functionally graded Ti-6Al-4V meshes fabricated by electron beam melting. Acta Mater, 150:1–15. https://doi.org/10.1016/j.actamat.2018.02.060
41. Dong Z, Zhao X, 2021, Application of TPMS structure in bone regeneration. Eng Regen, 2:154–162. https://doi.org/10.1016/J.ENGREG.2021.09.004
42. Liu F, Ran Q, Zhao M, et al., 2020, Additively manufactured continuous cell-size gradient porous scaffolds: Pore characteristics, mechanical properties and biological responses in vitro. Materials (Basel, Switzerland), 13:2589. https://doi.org/10.3390/MA13112589
43. Yadroitsava I, du Plessis A, Yadroitsev I, 2019, Bone regeneration on implants of titanium alloys produced by laser powder bed fusion: A review, in Titanium for Consumer Applications: Real World Use of Titanium, 197–233. https://doi.org/10.1016/B978-0-12-815820-3.00016-2
44. Bobyn JD, Pilliar RM, Cameron HU, et al., 1980, The optimum pore size for the fixation of porous surfaced metal implants by the ingrowth of bone. Clin Orthop Relat Res, 150:263–270. https://doi.org/10.1097/00003086-198007000-00045
45. Wang Z, Zhang M, Liu Z, et al., 2022, Biomimetic design strategy of complex porous structure based on 3D printing Ti-6Al-4V scaffolds for enhanced osseointegration. Mater Des, 218:110721. https://doi.org/10.1016/j.matdes.2022.110721
46. Taniguchi N, Fujibayashi S, Takemoto M, et al., 2016, Effect of pore size on bone ingrowth into porous titanium implants fabricated by additive manufacturing: An in vivo experiment. Mater Sci Eng C, 59:690–701. https://doi.org/10.1016/j.msec.2015.10.069
47. Mahmoud D, Elbestawi MA, 2019, Selective laser melting of porosity graded lattice structures for bone implants. Int J Adv Manuf Technol, 100:2915–2927. https://doi.org/10.1007/s00170-018-2886-9
48. Surmeneva MA, Surmenev RA, Chudinova EA, et al., 2017, Fabrication of multiple-layered gradient cellular metal scaffold via electron beam melting for segmental bone reconstruction. Mater Des, 133:195–204. https://doi.org/10.1016/j.matdes.2017.07.059
49. Tüzemen MÇ, Salamcı E, Ünal R, 2022, Additive manufacturing design approach to strut-based functionally graded porous structures for personalized implants. J Manuf Process, 84:1526–1540. https://doi.org/10.1016/J.JMAPRO.2022.10.045
50. Tüzemen MÇ, Salamcı E, Ünal R, 2022, Investigation of the relationship between flexural modulus of elasticity and functionally graded porous structures manufactured by AM. Mater Today Commun, 31:103592. https://doi.org/10.1016/J.MTCOMM.2022.103592
51. Murat Dursun A, Çağrı Tüzemen M, Salamci E, et al., 2022, Investigation of compatibility between design and additively manufactured parts of functionally graded porous structures. J Polytech, 25:1069–1082. https://doi.org/10.2339/POLITEKNIK.891080
52. Kelly CN, Wang T, Crowley J, et al., 2021, High-strength, porous additively manufactured implants with optimized mechanical osseointegration. Biomaterials, 279:121206. https://doi.org/10.1016/J.BIOMATERIALS.2021.121206
53. Yang L, Wu S, Yan C, et al., 2021, Fatigue properties of Ti-6Al-4V Gyroid graded lattice structures fabricated by laser powder bed fusion with lateral loading. Addit Manuf, 46:102214. https://doi.org/10.1016/J.ADDMA.2021.102214
54. Kelly CN, Wang T, Crowley J, et al., 2021, High-strength, porous additively manufactured implants with optimized mechanical osseointegration. Biomaterials, 279:121206. https://doi.org/10.1016/j.biomaterials.2021.121206
55. Zhang J, Chen X, Sun Y, et al., 2022, Design of a biomimetic graded TPMS scaffold with quantitatively adjustable pore size. Mater Des, 218:110665. https://doi.org/10.1016/J.MATDES.2022.110665
56. de Galarreta SR, Doyle RJ, Jeffers J, et al., 2021, Laser powder bed fusion of porous graded structures: A comparison between computational and experimental analysis. J Mech Behav Biomed Mater, 123:104784. https://doi.org/10.1016/J.JMBBM.2021.104784
57. Hildebrand T, Rüegsegger P, 1997, A new method for the model-independent assessment of thickness in three-dimensional images. J Microsc, 185:67–75. https://doi.org/10.1046/J.1365-2818.1997.1340694.X
58. Kolken HMA, Garcia AF, Du Plessis A, et al., 2021, Fatigue performance of auxetic meta-biomaterials. Acta Biomater, 126:511–523. https://doi.org/10.1016/j.actbio.2021.03.015
59. ASTM-E407 | Standard Practice for Microetching Metals and Alloys | Document Center, Inc.
60. ISO - ISO 13314:2011 - Mechanical testing of metals— Ductility testing—Compression test for porous and cellular metals.
61. Benedetti M, Klarin J, Johansson F, et al., 2019, Study of the compression behaviour of Ti6Al4V trabecular structures produced by additive laser manufacturing. Materials (Basel), 12:1471. https://doi.org/10.3390/MA12091471
62. Benedetti M, Galeotti F, Emanuelli L, et al., 2019, Deformation behaviour of Ti-6Al-4V trabecular structures produced by additive laser manufacturing. Euro PM 2019 Congr Exhib.
63. Schmitz A, Horst P, 2014, A finite element unit-cell method for homogenised mechanical properties of heterogeneous plates. Compos Part A Appl Sci Manuf, 61:23–32. https://doi.org/10.1016/J.COMPOSITESA.2014.01.014
64. Alwattar TA, Mian A, 2019, Development of an elastic material model for BCC lattice cell structures using finite element analysis and neural networks approaches. J Compos Sci, 3:33. https://doi.org/10.3390/JCS3020033
65. Kim HS, Al-Hassani STS, 2003, Effective elastic constants of two-dimensional cellular materials with deep and thick cell walls. Int J Mech Sci, 45:1999–2016. https://doi.org/10.1016/J.IJMECSCI.2004.02.002
66. Webb DC, Kormi K, Al-Hassani STS, 1995, Use of FEM in performance assessment of perforated plates subject to general loading conditions. Int J Press Vessel Pip, 64: 137–152. https://doi.org/10.1016/0308-0161(94)00078-W
67. Murchio S, Dallago M, Zanini F, et al., 2021, Additively manufactured Ti–6Al–4V thin struts via laser powder bed fusion: Effect of building orientation on geometrical accuracy and mechanical properties. J Mech Behav Biomed Mater, 119:104495. https://doi.org/10.1016/J.JMBBM.2021.104495
68. Gordon JV, Narra SP, Cunningham RW, et al., 2020, Defect structure process maps for laser powder bed fusion additive manufacturing. Addit Manuf, 36:101552. https://doi.org/10.1016/J.ADDMA.2020.101552
69. Abdulhadi HS, Mian A, 2019, Effect of strut length and orientation on elastic mechanical response of modified body-centered cubic lattice structures. Proc Inst Mech Eng Part L: J Mater Des Appl, 233:2219–2233. https://doi.org/10.1177/1464420719841084
70. Alkhatib SE, Tarlochan F, Mehboob H, et al., 2019, Finite element study of functionally graded porous femoral stems incorporating body-centered cubic structure. Artif Organs, 43:E152–E164. https://doi.org/10.1111/AOR.13444
71. Yang L, Li Y, Wu S, et al., 2022, Tailorable and predictable mechanical responses of additive manufactured TPMS lattices with graded structures. Mater Sci Eng A, 843:143109. https://doi.org/10.1016/J.MSEA.2022.143109
72. Lv Y, Wang B, Liu G, et al., 2022, Design of bone-like continuous gradient porous scaffold based on triply periodic minimal surfaces. J Mater Res Technol, 21:3650–3665. https://doi.org/10.1016/J.JMRT.2022.10.160
73. Belda R, Megías R, Marco M, et al., 2023, Numerical analysis of the influence of triply periodic minimal surface structures morphometry on the mechanical response. Comput Methods Programs Biomed, 230:107342. https://doi.org/10.1016/J.CMPB.2023.107342