Manufacturability of functionally graded porous β-Ti21S auxetic architected biomaterials produced by laser powder bed fusion: Comparison between 2D and 3D metrological characterization
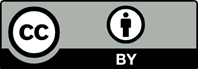
Functionally graded porous structures (FGPSs) are attracting increasing interest in the manufacture of prostheses that benefit from lower stiffness and optimized pore size for osseointegration. In this work, we explore the possibility of employing FGPSs with auxetic unit cells. Their negative Poisson’s ratio was exploited to reduce the loss of connection between prosthesis and bone usually occurring in standard implant loaded under tension and therefore undergoing lateral shrinking. In addition, to further improve osseointegration and mitigate stress shielding effects, auxetic FGPSs were fabricated in this work using a novel β-Ti21S alloy characterized by a lower Young’s modulus compared to traditional α + β Ti alloys. Specifically, two different auxetic FGPSs with aspect ratio equal to 1.5 and angle θ of 15° and 25° with a relative density (ρr ) gradient of 0.34, 0.49, 0.66 and of 0.40, 0.58, 0.75 were designed and printed by laser powder bed fusion. The 2D and 3D metrological characterization of the as-manufactured structures was compared with the design. 2D metrological characterization was carried out using scanning electron microscopy analysis, while for the 3D characterization, X-ray micro-CT imaging was used. An undersizing of the pore size and strut thickness in the as-manufactured sample was observed in both auxetic FGPSs. A maximum difference in the strut thickness of −14 and −22% was obtained in the auxetic structure with θ = 15° and 25°, respectively. On the contrary, a pore undersizing of −19% and −15% was evaluated in auxetic FGPS with θ = 15° and 25°, respectively. Compression mechanical tests allowed to determine stabilized elastic modulus of around 4 GPa for both FGPSs. Homogenization method and analytical equation were used and the comparison with experimental data highlights a good agreement of around 4% and 24% for θ = 15° and 25°, respectively.
1. Geetha M, Singh AK, Asokamani R, et al., 2009, Ti based biomaterials, the ultimate choice for orthopaedic implants-a review. Prog Mater Sci, 54: 397–425. https://doi.org/10.1016/j.pmatsci.2008.06.004
2. Gepreel MA, Niinomi M, 2013, Biocompatibility of Ti-alloys for long-term implantation. J Mech Behav Biomed Mater, 20: 407–415. https://doi.org/10.1016/j.jmbbm.2012.11.014
3. Kumar A, Nune KC, Misra RD, 2016, Biological functionality and mechanistic contribution of extracellular matrix-ornamented three dimensional Ti-6Al-4V mesh scaffolds. J Biomed Mater Res Part A, 104: 2751–2763. https://doi.org/10.1002/jbm.a.35809
4. Wang X, Xu S, Zhou S, et al., 2016, Topological design and additive manufacturing of porous metals for bone scaffolds and orthopaedic implants: A review. Biomaterials, 83: 127–141. https://doi.org/10.1016/j.biomaterials.2016.01.012
5. International Organization for Standardization, 1999, ISO 5832-2:1999, Implants for Surgery-metallic Materials- Part 2: Unalloyed titanium. International Organization for Standardization, Geneva, Switzerland. p3.
6. International Organization for Standardization, (n.d.), ISO 5832-14:2019-implants for Surgery-metallic Materials- Part 14: Wrought Titanium 15-molybdenum 5-zirconium 3-aluminium alloy. International Organization for Standardization, Geneva, Switzerland.
7. Materials Properties Handbook, (n.d.), Titanium Alloys. ASM International, Almere, Netherlands. Available from: https://www.asminternational.org/materials-resources/ results/-/journal_content/56/10192/06005g/publication [Last accessed on 2022 Jul 13].
8. ASTM, (n.d.), F1295-16-standard Specification for Wrought Titanium-6Aluminum-7Niobium Alloy for Surgical Implant Applications (UNS R56700). ASTM International, Pennsylvania.
9. ASTM, (n.d.), F3046-21-standard Specification for Wrought Titanium-3Aluminum-2.5Vanadium Alloy for Surgical Implant Applications (UNS R56320). ASTM International, Pennsylvania.
10. ASTM, (n.d.), F2066-18-standard Specification for Wrought Titanium-15 Molybdenum Alloy for Surgical Implant Applications (UNS R58150). ASTM International, Pennsylvania.
11. ASTM, (n.d.), F1813-21-standard Specification for Wrought Titanium-12Molybdenum-6Zirconium-2Iron Alloy for Surgical Implant (UNS R58120). ASTM International, Pennsylvania.
12. Polozov I, Sufiiarov A, Popovich A, et al., 2018, Synthesis of Ti-5Al, Ti-6Al-7Nb, and Ti-22Al-25Nb alloys from elemental powders using powder-bed fusion additive manufacturing. J Alloys Compd, 763: 436–445. https://doi.org/10.1016/j.jallcom.2018.05.325
13. Bolzoni L, Ruiz-Navas EM, Gordo E, 2014, On the microstructure and properties of the Ti-3Al-2.5V alloy obtained by powder metallurgy. In: TMS 2014 143rd Annual Meeting and Exhibiton. Springer, Champaign. p121–128. https://doi.org/10.1007/978-3-319-48237-8_17
14. Brunke F, Siemers C, Rösler J, 2020, Second-generation titanium alloys Ti-15Mo and Ti-13Nb-13Zr: A comparison of the mechanical properties for implant applications, MATEC Web Conf. 321: 05006. https://doi.org/10.1051/matecconf/202032105006
15. Duan R, Li S, Cai B, et al., 2021, A high strength and low modulus metastable β Ti-12Mo-6Zr-2Fe alloy fabricated by laser powder bed fusion in-situ alloying. Addit Manuf, 37: 101708. https://doi.org/10.1016/j.addma.2020.101708
16. Claros CA, Campanelli LC, Jorge AM, et al., 2021, Corrosion behaviour of biomedical β-titanium alloys with the surface-modified by chemical etching and electrochemical methods. Corros Sci, 188: 109544. https://doi.org/10.1016/j.corsci.2021.109544
17. Macias-Sifuentes MA, Xu C, Sanchez-Mata O, et al., 2021, Microstructure and mechanical properties of β-21S Ti alloy fabricated through laser powder bed fusion. Prog Addit Manuf, 6: 417–430. https://doi.org/10.1007/s40964-021-00181-7
18. Pellizzari M, Jam A, Tschon M, et al., 2020, A 3D-printed ultra-low young’s modulus β-Ti alloy for biomedical applications. Materials (Basel), 13: 2792. https://doi.org/10.3390/ma13122792
19. Jam A, du Plessis A, Lora C, et al., Manufacturability of lattice structures fabricated by laser powder bed fusion: A novel biomedical application of the beta Ti-21S alloy. Addit Manuf, 50: 102556. https://doi.org/10.1016/J.ADDMA.2021.102556
20. Gibson LJ, Ashby MF, Harley BA, (n.d.), Cellular Materials in Nature and Medicine. Cambridge University Press, Cambridge, United Kingdom. p309.
21. Ashby MF, 2005, The properties of foams and lattices. Philos Trans A Math Phys Eng Sci., 364: 15–30. https://doi.org/10.1098/RSTA.2005.1678
22. Benedetti M, du Plessis A, Ritchie RO, et al., 2021, Architected cellular materials: A review on their mechanical properties towards fatigue-tolerant design and fabrication. Mater Sci Eng R Rep, 144: 100606. https://doi.org/10.1016/j.mser.2021.100606
23. Zadpoor AA, 2019, Mechanical performance of additively manufactured meta-biomaterials. Acta Biomater, 85: 41–59. https://doi.org/10.1016/j.actbio.2018.12.038
24. Kolken HM, Janbaz S, Leeflang SM, et al., 2018, Rationally designed meta-implants: A combination of auxetic and conventional meta-biomaterials. Mater Horizons, 5: 28–35. https://doi.org/10.1039/c7mh00699c
25. Albertini F, Dirrenberger J, Sollogoub C, et al., 2021, Experimental and computational analysis of the mechanical properties of composite auxetic lattice structures. Addit Manuf, 47: 102351. https://doi.org/10.1016/j.addma.2021.102351
26. Kolken HM, Garcia AF, Du Plessis A, et al., 2021, Fatigue performance of auxetic meta-biomaterials. Acta Biomater, 126: 511–523. https://doi.org/10.1016/j.actbio.2021.03.015
27. Kolken HM, Zadpoor AA, 2017, Auxetic mechanical metamaterials. RSC Adv, 7: 5111–5129. https://doi.org/10.1039/c6ra27333e
28. Kolken HM, Lietaert K, van der Sloten T, et al., 2020, Mechanical performance of auxetic meta-biomaterials. J Mech Behav Biomed Mater, 104: 103658. https://doi.org/10.1016/j.jmbbm.2020.103658
29. Nečemer B, Kramberger J, Vuherer T, et al., 2019, Fatigue crack initiation and propagation in re-entrant auxetic cellular structures. Int J Fatigue, 126: 241–247. https://doi.org/10.1016/j.ijfatigue.2019.05.010
30. Mizzi L, Spaggiari A, 2021, Chiralisation of euclidean polygonal tessellations for the design of new auxetic metamaterials. Mech Mater, 153: 103698. https://doi.org/10.1016/j.mechmat.2020.103698
31. Yadroitsava I, du Plessis A, Yadroitsev I, 2019, Bone regeneration on implants of titanium alloys produced by laser powder bed fusion: A review. In: Titanium for Consumer Applications Real-world Use Titanium. Elsevier Publishing Company, Amsterdam, Netherlands. p197–233. https://doi.org/10.1016/B978-0-12-815820-3.00016-2
32. Shi J, Zhu L, Li L, et al., 2018, A TPMS-based method for modeling porous scaffolds for bionic bone tissue engineering. Sci Rep, 8: 7395. https://doi.org/10.1038/s41598-018-25750-9
33. Mahmoud D, Elbestawi MA, 2019, Selective laser melting of porosity graded lattice structures for bone implants. Int J Adv Manuf Technol, 100: 2915–2927. https://doi.org/10.1007/s00170-018-2886-9
34. Zhao S, Li SJ, Wang SG, et al., 2018, Compressive and fatigue behavior of functionally graded Ti-6Al-4V meshes fabricated by electron beam melting. Acta Mater, 150: 1–15. https://doi.org/10.1016/j.actamat.2018.02.060
35. Surmeneva MA, Surmenev RA, Chudinova EA, et al., 2017, Fabrication of multiple-layered gradient cellular metal scaffold via electron beam melting for segmental bone reconstruction. Mater Des, 133: 195–204. https://doi.org/10.1016/j.matdes.2017.07.059
36. Dursun AM, Tüzemen MC, Salamci E, et al., 2022, Investigation of compatibility between design and additively manufactured parts of functionally graded porous structures. J Polytech, 25: 1069–1082. https://doi.org/10.2339/politeknik.891080
37. Tüzemen MC, Salamcı E, Ünal R, 2022, Investigation of the relationship between flexural modulus of elasticity and functionally graded porous structures manufactured by AM. Mater Today Commun, 31: 103592. https://doi.org/10.1016/j.mtcomm.2022.103592
38. De Galarreta SR, Doyle RJ, Jeffers J, et al., Laser powder bed fusion of porous graded structures: A comparison between computational and experimental analysis. J Mech Behav Biomed Mater, 123: 104784. https://doi.org/10.1016/j.jmbbm.2021.104784
39. Liu YJ, Wang HL, Li SJ, et al., 2017, Compressive and fatigue behavior of beta-type titanium porous structures fabricated by electron beam melting. Acta Mater, 126: 58–66. https://doi.org/10.1016/j.actamat.2016.12.052
40. Luo JP, Huang YJ, Xu JY, et al., 2020, Additively manufactured biomedical Ti-Nb-Ta-Zr lattices with tunable Young’s modulus: Mechanical property, biocompatibility, and proteomics analysis. Mater Sci Eng C Mater Biol Appl, 114: 110903. https://doi.org/10.1016/j.msec.2020.110903
41. Gordon JV, Narra SP, Cunningham RW, et al., 2020, Defect structure process maps for laser powder bed fusion additive manufacturing. Addit Manuf, 36: 101552. https://doi.org/10.1016/J.ADDMA.2020.101552
42. Tang M, Pistorius PC, Beuth JL, 2017, Prediction of lack-of-fusion porosity for powder bed fusion. Addit Manuf, 14: 39–48. https://doi.org/10.1016/j.addma.2016.12.001
43. Zhang B, Li Y, Bai Q, 2017, Defect formation mechanisms in selective laser melting: A review. Chin J Mech Eng, 30: 515–527. https://doi.org/10.1007/S10033-017-0121-5
44. Alaña M, Cutolo A, Probst G, et al., 2020, Understanding elastic anisotropy in diamond based lattice structures produced by laser powder bed fusion: Effect of manufacturing deviations. Mater Des, 195: 108971. https://doi.org/10.1016/j.matdes.2020.108971
45. Hildebrand T, Rüegsegger P, 1997, A new method for the model-independent assessment of thickness in three-dimensional images. J Microsc, 185: 67–75. https://doi.org/10.1046/J.1365-2818.1997.1340694.X
46. ASTM, (n.d.), E407 Standard Practice for Microetching Metals and Alloys. United States, Document Center, Inc.
47. International Organization for Standardization, (n.d.), ISO 13314:2011-mechanical Testing of Metals-ductility Testing-compression Test for Porous and Cellular Metals. International Organization for Standardization, Geneva, Switzerland.
48. Benedetti M, Klarin J, Johansson F, et al., 2019, Study of the compression behaviour of Ti6Al4V trabecular structures produced by additive laser manufacturing. Materials (Basel), 12: 1471. https://doi.org/10.3390/MA12091471
49. Schmitz A, Horst P, 2014, A finite element unit-cell method for homogenised mechanical properties of heterogeneous plates. Compos Part A Appl Sci Manuf, 61: 23–32. https://doi.org/10.1016/j.compositesa.2014.01.014
50. Alwattar TA, Mian A, 2019, Development of an elastic material model for BCC lattice cell structures using finite element analysis and neural networks approaches. J Compos Sci, 3: 33. https://doi.org/10.3390/jcs3020033
51. Kim HS, Al-Hassani ST, 2003, Effective elastic constants of two-dimensional cellular materials with deep and thick cell walls. Int J Mech Sci, 45: 1999–2016. https://doi.org/10.1016/j.ijmecsci.2004.02.002
52. Webb DC, Kormi K, Al-Hassani ST, 1995, Use of FEM in performance assessment of perforated plates subject to general loading conditions. Int J Press Vessel Pip, 64: 137–152. https://doi.org/10.1016/0308-0161(94)00078-W
53. Yang L, Harrysson O, West H, et al., 2015, Mechanical properties of 3D re-entrant honeycomb auxetic structures realized via additive manufacturing. Int J Solids Struct, 69–70: 475–490. https://doi.org/10.1016/j.ijsolstr.2015.05.005
54. Murchio S, Dallago M, Zanini F, et al., 2021, Additively manufactured Ti-6Al-4V thin struts via laser powder bed fusion: Effect of building orientation on geometrical accuracy and mechanical properties. J Mech Behav Biomed Mater, 119: 104495. https://doi.org/10.1016/j.jmbbm.2021.104495
55. Yuan L, Ding S, Wen C, 2019, Additive manufacturing technology for porous metal implant applications and triple minimal surface structures: A review. Bioact Mater, 4: 56–70. https://doi.org/10.1016/j.bioactmat.2018.12.003
56. Deshpande VS, Ashby MF, Fleck NA, 2001, Foam topology: Bending versus stretching dominated architectures. Acta Mater, 49: 1035–1040. https://doi.org/10.1016/S1359-6454(00)00379-7
57. Wang AJ, McDowell DL, 2004, In-plane stiffness and yield strength of periodic metal honeycombs. J Eng Mater Technol, 126: 137–156. https://doi.org/10.1115/1.1646165