Computational simulation-based comparative analysis of standard 3D printing and conical nozzles for pneumatic and piston-driven bioprinting
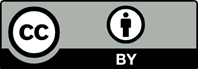
Bioprinting is an application of additive manufacturing that can deliver promising results in regenerative medicine. Hydrogels, as the most used materials in bioprinting, are experimentally analyzed to assure printability and suitability for cell culture. Besides hydrogel features, the inner geometry of the microextrusion head might have an equal impact not only on printability but also on cellular viability. In this regard, standard 3D printing nozzles have been widely studied to reduce inner pressure and get faster printings using highly viscous melted polymers. Computational fluid dynamics is a useful tool capable of simulating and predicting the hydrogel behavior when the extruder inner geometry is modified. Hence, the objective of this work is to comparatively study the performance of a standard 3D printing and conical nozzles in a microextrusion bioprinting process through computational simulation. Three bioprinting parameters, namely pressure, velocity, and shear stress, were calculated using the level-set method, considering a 22G conical tip and a 0.4 mm nozzle. Additionally, two microextrusion models, pneumatic and piston-driven, were simulated using dispensing pressure (15 kPa) and volumetric flow (10 mm3 /s) as input, respectively. The results showed that the standard nozzle is suitable for bioprinting procedures. Specifically, the inner geometry of the nozzle increases the flow rate, while reducing the dispensing pressure and maintaining similar shear stress compared to the conical tip commonly used in bioprinting.
1. Cambridge dictionary, 2023, Bioprinting Definition, viewed on January 23, 2023. (n.d.). https://dictionary.cambridge.org/es/diccionario/ingles/ bioprinting
2. Ng WL, Chua CK, Shen YF, 2019, Print me an organ! Why we are not there yet. Progr Polym Sci, 97:101145. https://doi.org/10.1016/j.progpolymsci.2019.101145
3. Li S, Liu S, Wang X, 2022, Advances of 3D printing in vascularized organ construction. Int J Bioprint, 8(3):588. https://doi.org/10.18063/ijb.v8i3.588
4. Pedroza-González SC, Rodriguez-Salvador M, Pérez-Benítez BE, et al., 2021, Bioinks for 3D bioprinting: A scientometric analysis of two decades of progress. Int J Bioprint, 7(2):337. https://doi.org/10.18063/ijb.v7i2.337
5. Ng WL, Lee JM, Zhou M, et al., 2020, Vat polymerization-based bioprinting—Process, materials, applications and regulatory challenges. Biofabrication, 12(2):022001. https://doi.org/10.1088/1758-5090/ab6034
6. Ozbolat IT, Hospodiuk M, 2016, Current advances and future perspectives in extrusion-based bioprinting. Biomaterials, 76:321–343. https://doi.org/10.1016/j.biomaterials.2015.10.076
7. Yilmaz B, Al Rashid A, Mou YA, et al., 2021, Bioprinting: A review of processes, materials and applications. Bioprinting, 23:e00148. https://doi.org/10.1016/j.bprint.2021.e00148
8. Li Q, Zhang B, Xue Q, et al., 2021, A systematic thermal analysis for accurately predicting the extrusion printability of alginate-gelatin-based hydrogel bioinks. Int J Bioprint, 7(3):394. https://dx.doi.org/10.18063/ijb.v7i3.394
9. Fu E, Wentland L, 2022, A survey of 3D printing technology applied to paper microfluidics. Lab Chip, 22(1):9–25. https://doi.org/10.1039/D1LC00768H
10. Li X, Liu B, Pei B, et al., 2020, Inkjet bioprinting of biomaterials. Chem Rev, 120(19):10793–10833. https://doi.org/10.1021/acs.chemrev.0c00008
11. Heinrich MA, Liu W, Jimenez A, et al., 2019, 3D bioprinting: From benches to translational applications. Small, 15(23):1805510. https://doi.org/10.1002/smll.201805510
12. Singh, AV, Dad Ansari M, Wang S, et al., 2019, The adoption of three-dimensional additive manufacturing from biomedical material design to 3D organ printing. Appl Sci, 9(4):811. https://doi.org/10.3390/app9040811
13. Chaudhry A, Naheed A, Latif Z, et al., 2022, Applications and limitations of 3D bioprinters in tissue culturing: A review. Abasyn J Life Sci, 5(1):31–43. https://doi.org/10.34091/AJLS.5.1.4
14. Ng WL, Huang X, Shkolnikov V, et al., 2022, Controlling droplet impact velocity and droplet volume: Key factors to achieving high cell viability in sub-nanoliter droplet-based bioprinting. Int J Bioprint, 8(1):424. https://dx.doi.org/10.18063/ijb.v8i1.424
15. Gómez-Blanco JC, Galván-Chacón V, Patrocinio D, et al., 2021, Improving cell viability and velocity in μ-extrusion bioprinting with a novel pre-incubator bioprinter and a standard FDM 3D printing nozzle. Materials, 14(11):3100. https://doi.org/10.3390/ma14113100
16. Fu Z, Naghieh S, Xu C, et al., 2021, Printability in extrusion bioprinting. Biofabrication, 13(3):033001. https://doi.org/10.1088/1758-5090/abe7ab
17. Gao T, Gillispie GJ, Copus JS, et al., 2018, Optimization of gelatin–alginate composite bioink printability using rheological parameters: A systematic approach. Biofabrication, 10(3):034106. https://doi.org/10.1088/1758-5090/aacdc7
18. He Y, Yang F, Zhao H, et al., 2016, Research on the printability of hydrogels in 3D bioprinting. Sci Rep, 6(1):1–13. https://doi.org/10.1038/srep29977
19. Jeon O, Lee YB, Hinton TJ, et al., 2019, Cryopreserved cell-laden alginate microgel bioink for 3D bioprinting of living tissues. Mater Today Chem, 12:61–70. https://doi.org/10.1016/j.mtchem.2018.11.009
20. Yang RM, Xu J, Huang CC, 2022, Effect of ionic crosslinking on morphology and thermostability of biomimetic supercritical fluids-decellularized dermal-based composite bioscaffolds for bioprinting applications. Int J Bioprint. 9(1):625. https://dx.doi.org/10.18063/ijb.v9i1.625
21. Raddatz L, Lavrentieva A, Pepelanova I, et al., 2018, Development and application of an additively manufactured calcium chloride nebulizer for alginate 3D-bioprinting purposes. J Funct Biomater, 9(4):63. https://doi.org/10.3390/jfb9040063
22. Zheng Z, Wu J, Liu M, et al., 2018, 3D bioprinting of self‐standing silk‐based bioink. Adv Healthc Mater, 7(6):1701026. https://doi.org/10.1002/adhm.201701026
23. Wu D, Yu Y, Tan J, et al., 2018, 3D bioprinting of gellan gum and poly (ethylene glycol) diacrylate based hydrogels to produce human-scale constructs with high-fidelity. Mater Design, 160:486–495. https://doi.org/10.1016/j.matdes.2018.09.040
24. Yan KC, Paluch K, Nair K, et al., 2009, Effects of process parameters on cell damage in a 3d cell printing process. In ASME International Mechanical Engineering Congress and Exposition (Vol. 43758, pp. 75–81). https://doi.org/10.1115/IMECE2009-11528
25. Li M, Tian X, Kozinski JA, et al., 2015, Modeling mechanical cell damage in the bioprinting process employing a conical needle. J Mech Med Biol, 15(05):1550073. https://doi.org/10.1142/S0219519415500736.
26. Boularaoui S, Al Hussein G, Khan KA, et al., 2020, An overview of extrusion-based bioprinting with a focus on induced shear stress and its effect on cell viability. Bioprinting, 20:e00093. https://doi.org/10.1016/j.bprint.2020.e00093
27. Mancha Sánchez E, Gómez-Blanco JC, López Nieto E, et al., 2020, Hydrogels for bioprinting: A systematic review of hydrogels synthesis, bioprinting parameters, and bioprinted structures behavior. Front Bioeng Biotechnol, 8:776. https://doi.org/10.3389/fbioe.2020.00776
28. Axpe E, Oyen ML, 2016, Applications of alginate-based bioinks in 3D bioprinting. Int J Mol Sci, 17(12):1976. https://doi.org/10.3390/ijms17121976
29. Dutta S, Cohn D, 2017, Temperature and pH responsive 3D printed scaffolds. J Mater Chem B, 5(48):9514–9521. https://doi.org/10.1039/c7tb02368e
30. Kim W, Kim G, 2020, 3D bioprinting of functional cell-laden bioinks and its application for cell-alignment and maturation. Appl Mater Today, 19:100588. https://doi.org/10.1016/j.apmt.2020.100588
31. Zhou D, Chen J, Liu B, et al., 2019, Bioinks for jet-based bioprinting. Bioprinting, 16:e00060. https://doi.org/10.1016/j.bprint.2019.e00060
32. Ouyang L, Yao R, Zhao Y, et al., 2016, Effect of bioink properties on printability and cell viability for 3D bioplotting of embryonic stem cells. Biofabrication, 8(3):035020. https://doi.org/10.1088/1758-5090/8/3/035020
33. Kiyotake EA, Douglas AW, Thomas EE, et al., 2019, Development and quantitative characterization of the precursor rheology of hyaluronic acid hydrogels for bioprinting. Acta Biomater, 95:176–187. https://doi.org/10.1016/j.actbio.2019.01.041
34. Jia J, Richards DJ, Pollard S, et al., 2014, Engineering alginate as bioink for bioprinting. Acta Biomater, 10(10):4323–4331. https://doi.org/10.1016/j.actbio.2014.06.034
35. Ashammakhi N, Ahadian S, Xu C, et al., 2019, Bioinks and bioprinting technologies to make heterogeneous and biomimetic tissue constructs. Mater Today Biol, 1:100008. https://doi.org/10.1016/j.mtbio.2019.100008
36. Zhang S, Vijayavenkataraman S, Lu WF, et al., 2019, A review on the use of computational methods to characterize, design, and optimize tissue engineering scaffolds, with a potential in 3D printing fabrication. J Biomed Mater Res Part B Appl Biomater, 107(5):1329–1351. https://doi.org/10.1002/jbm.b.34226
37. Göhl J, Markstedt K, Mark A, et al., 2018, Simulations of 3D bioprinting: Predicting bioprintability of nanofibrillar inks. Biofabrication, 10(3):034105. https://doi.org/10.1088/1758-5090/aac872
38. Blaeser A, Duarte-Campos DF, Puster U, et al., 2016, Controlling shear stress in 3D bioprinting is a key factor to balance printing resolution and stem cell integrity. Adv Healthc Mater, 5(3):326–333. https://doi.org/10.1002/adhm.201500677
39. Liu W, Heinrich MA, Zhou Y, et al., 2017, Extrusion bioprinting of shear‐thinning gelatin methacryloyl bioinks. Adv Healthc Mater, 6(12):1601451. https://doi.org/10.1002/adhm.201601451
40. Magalhães IP, Oliveira PMD, Dernowsek J, et al., 2019. Investigation of the effect of nozzle design on rheological bioprinting properties using computational fluid dynamics. Matéria (Rio J.), 24(3):12401. https://doi.org/10.1590/s1517-707620190003.0714
41. Martanto W, Baisch SM, Costner EA, et al., 2005, Fluid dynamics in conically tapered microneedles. AIChE J, 51(6):1599–1607. https://doi.org/10.1002/aic.10424
42. Reid JA, Mollica PA, Johnson GD, et al., 2016, Accessible bioprinting: Adaptation of a low-cost 3D-printer for precise cell placement and stem cell differentiation. Biofabrication, 8(2):025017. https://doi.org/10.1088/1758-5090/8/2/025017
43. Leppiniemi J, Lahtinen P, Paajanen A, et al., 2017, 3D-printable bioactivated nanocellulose–alginate hydrogels. ACS Appl Mater Interfaces, 9(26):21959–21970. https://doi.org/10.1021/acsami.7b02756
44. Nair K, Yan KC, Sun W, 2008, A computational modeling approach for the characterization of mechanical properties of 3D alginate tissue scaffolds. J Appl Biomater Biomech, 6(1):35–46. https://doi.org/10.1177/228080000800600106
45. Smith C, Oldt G, 2018, Multiaxial bio-printer head, viewed January 23, 2023, https://5f6357c8-abe2-426e-bc22-b9f609a0b347.filesusr.com/ugd/e69967_73cde5aebac44f11b0432814832a2110.pdf
46. Stewart B, 2017, 3D Bioprinting Hydrogel for Tissue Engineering an Ascending Aortic Scaffold, Thesis, Digital Commons @ DU, University of Denver.
47. Liravi F, Darleux R, Toyserkani E, 2017, Additive manufacturing of 3D structures with non-Newtonian highly viscous fluids: Finite element modeling and experimental validation. Addit Manuf, 13:113–123. https://doi.org/10.1016/j.addma.2016.10.008
48. Billiet T, Gevaert E, De Schryver T, et al., 2014, The 3D printing of gelatin methacrylamide cell-laden tissue-engineered constructs with high cell viability. Biomaterials, 35(1):49–62. https://doi.org/10.1016/j.biomaterials.2013.09.078
49. Samanipour R, Wang Z, Ahmadi A, et al., 2016, Experimental and computational study of microfluidic flow‐focusing generation of gelatin methacrylate hydrogel droplets. J Appl Polym Sci, 133(29):43701. https://doi.org/10.1002/app.43701
50. Gómez-Blanco JC, Mancha-Sánchez E, Marcos AC, et al., 2020, Bioink temperature influence on shear stress, pressure and velocity using computational simulation. Processes, 8(7):865. https://doi.org/10.3390/pr8070865
51. Verma A, Vishnoi P, Sukhotskiy V, et al., 2018, Numerical simulation of extrusion additive manufacturing: Fused deposition modelling, in TechConnect Briefs, 4, 118–121.
52. Serdeczny MP, Comminal R, Pedersen DB, et al., 2018, Experimental validation of a numerical model for the strand shape in material extrusion additive manufacturing. Addit Manuf, 24:145–153. https://doi.org/10.1016/j.addma.2018.09.022
53. E3D-ONLINE JR, 2016, E3D V6 series blueprint, viewed January 23, 2023, https://e3d-online.zendesk.com/hc/en-us/article_ attachments/4904924634141/V6-NOZZLE-ALL__6_.pdf
54. JB, 2019, Cellink bioink biopritning protocol, viewed January 23, 2023, https://www.cellink.com/wp-content/uploads/2023/02/ Bioprinting-Protocol-CELLINK-Bioink_2-Jan-2023-2.pdf
55. Müller M, Öztürk E, Arlov Ø, et al., 2017, Alginate sulfate– nanocellulose bioinks for cartilage bioprinting applications. Ann Biomed Eng, 45(1):210–223. https://doi.org/10.1007/s10439-016-1704-5