A hypothesis on the equilibrium between dopamine toxicity and detoxification: The roles of NQO2 and UDP-glucuronosyltransferases
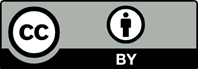
NQO2 and tyrosine hydroxylase are co-expressed in dopaminergic neurons. These neurons produce dopamine, a diol, which, under aerobic conditions, can spontaneously revert to the more stable form, the o-quinone. O-quinones are preferred substrates of NQO2 over p-quinones. In ad hoc conditions, NQO2 reduces o-quinones into the original diols, leading to a futile cycle, the endpoint of which is a strong local production of reactive oxygen species that is deadly for the cells. This futile cycle can be interrupted by the conjugation of dopamine with UDP-glucuronic acid, leading to a glucuronide that cannot be part of the cycle because the glucuronide is not a substrate of NQO2. In this paper, we confer whether this futile cycle could be one of the causes of the specific death of dopaminergic neuronal population that is the signature of some degenerative diseases.
Stich HF, Anders F, 1989, The involvement of reactive oxygen species in oral cancers of betel quid/tobacco chewers. Mutat Res, 214: 47–61. https://doi.org/10.1016/0027-5107(89)90197-8
Kamp DW, Graceffa P, Pryor WA, et al., 1992, The role of free radicals in asbestos-induced diseases. Free Radic Biol Med, 12: 293–315. https://doi.org/10.1016/0891-5849(92)90117-y
Hajam YA, Rani R, Ganie SY, et al., 2022, Oxidative stress in human pathology and aging: Molecular mechanisms and perspectives. Cells, 11: 552. https://doi.org/10.3390/cells11030552
Lü JM, Lin PH, Yao Q, et al., 2010, Chemical and molecular mechanisms of antioxidants: Experimental approaches and model systems. J Cell Mol Med, 14: 840–860. https://doi.org/10.1111/j.1582-4934.2009.00897.x
Nutter LM, Ngo EO, Fisher GR, et al., 1992, DNA strand scission and free radical production in menadione-treated cells. Correlation with cytotoxicity and role of NADPH quinone acceptor oxidoreductase. J Biol Chem, 267: 2474–2479. https://doi.org/10.1016/S0021-9258(18)45903-0
Qiu XB, Cadenas E, 1997, The role of NAD(P)H: Quinone oxidoreductase in quinone-mediated p21 induction in human colon carcinoma cells. Arch Biochem Biophys, 346: 241–251. https://doi.org/10.1006/abbi.1997.0329
Criddle DN, Gillies S, Baumgartner-Wilson HK, et al., 2006, Menadione-induced reactive oxygen species generation via redox cycling promotes apoptosis of murine pancreatic acinar cells. J Biol Chem, 281: 40485–40492. https://doi.org/10.1074/jbc.M607704200
Reybier K, Perio P, Ferry G, et al., 2011, Insights into the redox cycle of human quinone reductase 2. Free Radic Res, 45: 1184–1195. https://doi.org/10.3109/10715762.2011.605788
Cassagnes LE, Chhour M, Pério P, et al., 2018, Oxidative stress and neurodegeneration: The possible contribution of quinone reductase 2. Free Radic Biol Med, 120: 56–61. https://doi.org/10.1016/j.freeradbiomed.2018.03.002
Vella F, Ferry G, Delagrange P, et al., 2005, NRH: quinone reductase 2: An enzyme of surprises and mysteries. Biochem Pharmacol, 71: 1–12. https://doi.org/10.1016/j.bcp.2005.09.019
Janda E, Nepveu F, Calamini B, et al., 2020, Molecular pharmacology of NRH: Quinone oxidoreductase 2: a detoxifying enzyme acting as an undercover toxifying enzyme. Mol Pharmacol, 98: 620–33. https://doi.org/10.1124/molpharm.120.000105
Liao S, Dulaney JT, Williams-Ashman HG, 1962, Purification and properties of a flavoprotein catalyzing the oxidation of reduced ribosyl nicotinamide. J Biol Chem, 237: 2981–2987.
Zhao Q, Yang XL, Holtzclaw WD, et al., 1997, Unexpected genetic and structural relationships of a long-forgotten flavoenzyme to NAD(P)H: quinone reductase (DT-diaphorase). Proc Natl Acad Sci U S A, 94: 1669–1674. https://doi.org/10.1073/pnas.94.5.1669
Formentini L, Moroni F, Chiarugi A, 2009, Detection and pharmacological modulation of nicotinamide mononucleotide (NMN) in vitro and in vivo. Biochem Pharmacol, 77: 1612–1620. https://doi.org/10.1016/j.bcp.2009.02.017
Sonavane M, Hayat F, Makarov M, et al., 2020, Dihydronicotinamide riboside promotes cell-specific cytotoxicity by tipping the balance between metabolic regulation and oxidative stress. PLoS One, 15: e0242174. https://doi.org/10.1371/journal.pone.0242174
Makarov MV, Hayat F, Graves B, et al., 2021, Chemical and biochemical reactivity of the reduced forms of Nicotinamide riboside. ACS Chem Biol, 16: 604–614. https://doi.org/10.1021/acschembio.0c00757
Islam F, Leung KK, Walker MD, et al., 2022, The unusual cosubstrate specificity of NQO2: Conservation throughout the amniotes and implications for cellular function. Front Pharmacol, 13: 838500. https://doi.org/10.3389/fphar.2022.838500
Boutin JA, Bouillaud F, Janda E, et al., 2019, S29434, a quinone reductase 2 inhibitor: Main biochemical and cellular characterization. Mol Pharmacol, 95: 269–285. https://doi.org/10.1124/mol.118.114231
Leung KKK, Shilton BH, 2015, Binding of DNA-intercalating agents to oxidized and reduced quinone reductase 2. Biochemistry, 54: 7438–7448. https://doi.org/10.1021/acs.biochem.5b00884
Cassagnes LE, Perio P, Ferry G, et al., 2015, In cellulo monitoring of quinone reductase activity and reactive oxygen species production during the redox cycling of 1,2 and 1,4 quinones. Free Radic Biol Med, 89: 126–134. https://doi.org/10.1016/j.freeradbiomed.2015.07.150
Crocker AD, 1994, Experimental and clinical pharmacology: Dopamine- mechanisms of action. Aust Prescr, 17: 17–21. https://doi.org/10.18773/austprescr.1994.023
Nasrolahi A, Javaherforooshzadeh F, Jafarzadeh- Gharehziaaddin M, et al., 2022, Therapeutic potential of neurotrophic factors in Alzheimer’s disease. Mol Biol Rep, 49: 2345–2357. https://doi.org/10.1007/s11033-021-06968-9
Grosset D, 2010, Therapy adherence issues in Parkinson’s disease. J Neurol Sci, 289: 115–118. https://doi.org/10.1016/j.jns.2009.08.053
Miyazaki I, Asanuma M, 2009, Approaches to prevent dopamine quinone-induced neurotoxicity. Neurochem Res, 34: 698–706. https://doi.org/10.1007/s11064-008-9843-1
Smythies JR, 1997, Oxidative reactions and schizophrenia: A review-discussion. Schizophr Res, 24: 357–364. https://doi.org/10.1016/s0920-9964(97)00005-4
Latif S, Jahangeer M, Maknoon Razia D, et al., 2021, Dopamine in Parkinson’s disease. Clin Chim Acta, 522: 114–126. https://doi.org/10.1016/j.cca.2021.08.009
Zhu L, Sun C, Ren J, et al., 2019, Stress-induced precocious aging in PD-patient iPSC-derived NSCs may underlie the pathophysiology of Parkinson’s disease. Cell Death Dis, 10: 105. https://doi.org/10.1038/s41419-019-1313-y
Best JA, Nijhout HF, Reed MC, 2009, Homeostatic mechanisms in dopamine synthesis and release: A mathematical model. Theor Biol Med Model, 6: 21. https://doi.org/10.1186/1742-4682-6-21
Asanuma M, Miyazaki I, Diaz-Corrales FJ, et al., 2004, Quinone formation as dopaminergic neuron-specific oxidative stress in the pathogenesis of sporadic Parkinson’s disease and neurotoxin-induced parkinsonism. Acta Med Okayama, 58: 221–233. https://doi.org/10.18926/AMO/32105
Miyazaki I, Asanuma M, 2008, Dopaminergic neuron-specific oxidative stress caused by dopamine itself. Acta Med Okayama, 62: 141–150. https://doi.org/10.18926/AMO/30942
Sulzer D, Zecca L, 2000, Intraneuronal dopamine-quinone synthesis: A review. Neurotox Res, 1: 181–195. https://doi.org/10.1007/BF03033289
Hattoria N, Wanga M, Taka H, et al., 2009, Toxic effects of dopamine metabolism in Parkinson’s disease. Parkinsonism Relat Disord, 15 Suppl 1: S35–S38. https://doi.org/10.1016/S1353-8020(09)70010-0
Boutin JA, 1987, Indirect evidences of UDP-glucuronosyltransferase heterogeneity: how can it help purification? Drug Metab Rev, 18: 517–551. https://doi.org/10.3109/03602538708994131
Jackson MR, Fournel-Gigleux S, Harding D, et al., 1988, Examination of the substrate specificity of cloned rat kidney phenol UDP-glucuronyltransferase expressed in COS-7 cells. Mol Pharmacol, 34: 638–642.
Testa B, Krämer SD, 2007, The biochemistry of drug metabolism an introduction: Part 2. Redox reactions and their enzymes. Chem Biodivers, 4: 257–405. https://doi.org/10.1002/cbdv.200790032
Renaud HJ, Cui JY, Khan M, et al., 2011, Tissue distribution and gender-divergent expression of 78 cytochrome P450 mRNAs in mice. Toxicol Sci, 124: 261–277. https://doi.org/10.1093/toxsci/kfr240
Tourancheau A, Rouleau M, Guauque-Olarte S, et al., 2018, Quantitative profiling of the UGT transcriptome in human drug-metabolizing tissues. Pharmacogenomics J, 18: 251–261. https://doi.org/10.1038/tpj.2017.5
Suleman FG, Ghersi-Egea JF, Leininger-Muller B, et al., 1993, Uridine diphosphate-glucuronosyltransferase activities in rat brain microsomes. Neurosci Lett, 161: 219–222. https://doi.org/10.1016/0304-3940(93)90298-y
Leclerc S, Heydel JM, Amossé V, et al., 2002, Glucuronidation of odorant molecules in the rat olfactory system: Activity, expression and age-linked modifications of UDP-glucuronosyltransferase isoforms, UGT1A6 and UGT2A1, and relation to mitral cell activity. Brain Res Mol Brain Res, 107: 201–213. https://doi.org/10.1016/s0169-328x(02)00455-2
King CD, Rios GR, Green MD, et al., 2000, UDP-glucuronosyltransferases. Curr Drug Metab, 1: 143–161. https://doi.org/10.2174/1389200003339171
Ghosh C, Hossain M, Puvenna V, et al., 2013, Expression and functional relevance of UGT1A4 in a cohort of human drug-resistant epileptic brains. Epilepsia, 54: 1562–1570. https://doi.org/10.1111/epi.12318
Ouzzine M, Gulberti S, Ramalanjaona N, et al., 2014, The UDP-glucuronosyltransferases of the blood-brain barrier: Their role in drug metabolism and detoxication. Front Cell Neurosci, 8: 349. https://doi.org/10.3389/fncel.2014.00349
Kutsuno Y, Hirashima R, Sakamoto M, et al., 2015, Expression of UDP-glucuronosyltransferase 1 (UGT1) and glucuronidation activity toward endogenous substances in humanized UGT1 mouse brain. Drug Metab Dispos, 43: 1071–1076. https://doi.org/10.1124/dmd.115.063719
El-Bachá RS, Leclerc S, Netter P, et al., 2000, Glucuronidation of apomorphine. Life Sci, 67: 1735–1745. https://doi.org/10.1016/s0024-3205(00)00764-5
Kilpatrick GJ, Smith TW, 2005, Morphine-6-glucuronide: Actions and mechanisms. Med Res Rev, 25: 521–544. https://doi.org/10.1002/med.20035
Landsberg L, Berardino MB, Stoff J, et al., 1978, Further studies on catechol uptake and metabolism in rat small bowel in vivo: (1) A quantitatively significant process with distinctive structural specifications; and (2) the formation of a dopamine glucuronide reservoir after chronic l-dopa feeding. Biochem Pharmacol, 27: 1365–1371. https://doi.org/10.1016/0006-2952(78)90121-1
Alexander N, Yoneda S, Vlachakis ND, et al., 1984, Role of conjugation and red blood cells for inactivation of circulating catecholamines. Am J Physiol, 247: R203–R207. https://doi.org/10.1152/ajpregu.1984.247.1.R203
Gaudin C, Ruget G, Selz F, et al., 1985, Free and conjugated catecholamines in digestive tissues of rats. Life Sci, 37: 1469–1474. https://doi.org/10.1016/0024-3205(85)90177-8
Berndt TJ, MacDonald A, Walikonis R, et al., 1993, Excretion of catecholamines and metabolites in response to increased dietary phosphate intake. J Lab Clin Med, 122: 80–84.
Azoui R, Schneider J, Dong WX, et al., 1997, Red blood cells participate in the metabolic clearance of catecholamines in the rat. Life Sci, 60: 357–367. https://doi.org/10.1016/s0024-3205(96)00659-5
Itäaho K, Court MH, Uutela P, et al., 2009, Dopamine is a low-affinity and high-specificity substrate for the human UDP-glucuronosyltransferase 1A10. Drug Metab Dispos, 37: 768–775. https://doi.org/10.1124/dmd.108.025692
Uutela P, Karhu L, Piepponen P, et al., 2009, Discovery of dopamine glucuronide in rat and mouse brain microdialysis samples using liquid chromatography tandem mass spectrometry. Anal Chem, 81: 427–434. https://doi.org/10.1021/ac801846w
Suominen T, Uutela P, Ketola RA, et al., 2013, Determination of serotonin and dopamine metabolites in human brain microdialysis and cerebrospinal fluid samples by UPLC-MS/ MS: Discovery of intact glucuronide and sulfate conjugates. PLoS One, 8: e68007. https://doi.org/10.1371/journal.pone.0068007
Wang PC, Kuchel O, Buu NT, et al., 1983, Catecholamine glucuronidation: An important metabolic pathway for dopamine in the rat. J Neurochem, 40: 1435–1440. https://doi.org/10.1111/j.1471-4159.1983.tb13587.x
Chhour M, Perio P, Gayon R, et al., 2021, Association of NQO2 with UDP-glucuronosyltransferases reduces menadione toxicity in neuroblastoma cells. Front Pharmacol, 12: 660641. https://doi.org/10.3389/fphar.2021.660641
Mailliet F, Ferry G, Vella F, et al., 2004, Organs from mice deleted for NRH: quinone oxidoreductase 2 are deprived of the melatonin binding site MT3. FEBS Lett, 578: 116–120. https://doi.org/10.1016/j.febslet.2004.10.083
Fu Y, Buryanovskyy L, Zhang Z, 2008, Quinone reductase 2 is a catechol quinone reductase. J Biol Chem, 283: 23829–23835. https://doi.org/10.1074/jbc.M801371200
Ross D, Kepa JK, Winski SL, et al., 2000, NAD(P)H: quinone oxidoreductase 1 (NQO1): Chemoprotection, bioactivation, gene regulation and genetic polymorphisms. Chem Biol Interact, 129: 77–97. https://doi.org/10.1016/s0009-2797(00)00199-x
Ross D, Siegel D, 2004, NAD(P)H: quinone oxidoreductase 1 (NQO1, DT-diaphorase), functions and pharmacogenetics. Methods Enzymol, 382: 115–144. https://doi.org/10.1016/S0076-6879(04)82008-1
Goodman RP, Calvo SE, Mootha VK, 2018, Spatiotemporal compartmentalization of hepatic NADH and NADPH metabolism. J Biol Chem, 293: 7508–7516. https://doi.org/10.1074/jbc.TM117.000258
Gould NL, Sharma V, Hleihil M, et al., 2020, Dopamine-dependent QR2 pathway activation in CA1 interneurons enhances novel memory formation. J Neurosci, 40: 8698–8714. https://doi.org/10.1523/JNEUROSCI.1243-20.2020
Gould NL, Elkobi A, Edry E, et al., 2020, Muscarinic-dependent miR-182 and QR2 expression regulation in the anterior insula enables novel taste learning. eNeuro, 7: ENEURO.0067-20.2020. https://doi.org/10.1523/ENEURO.0067-20.2020
Rappaport AN, Jacob E, Sharma V, et al., 2015, Expression of quinone reductase-2 in the cortex is a muscarinic acetylcholine receptor-dependent memory consolidation constraint. J Neurosci, 35: 15568–15581. https://doi.org/10.1523/JNEUROSCI.1170-15.2015
Hashimoto T, Nakai M, 2011, Increased hippocampal quinone reductase 2 in Alzheimer’s disease. Neurosci Lett, 502: 10–12. https://doi.org/10.1016/j.neulet.2011.07.008
Janda E, Lascala A, Carresi C, et al., 2015, Parkinsonian toxin-induced oxidative stress inhibits basal autophagy in astrocytes via NQO2/quinone oxidoreductase 2: Implications for neuroprotection. Autophagy, 11: 1063–1080. https://doi.org/10.1080/15548627.2015.1058683
Harada S, Fujii C, Hayashi A, et al., 2001, An association between idiopathic Parkinson’s disease and polymorphisms of phase II detoxification enzymes: Glutathione S-transferase M1 and quinone oxidoreductase 1 and 2. Biochem Biophys Res Commun, 288: 887–892. https://doi.org/10.1006/bbrc.2001.5868
Wang W, Le WD, Pan T, et al., 2008, Association of NRH: quinone oxidoreductase 2 gene promoter polymorphism with higher gene expression and increased susceptibility to Parkinson’s disease. J Gerontol A Biol Sci Med Sci, 63: 127–134. https://doi.org/10.1093/gerona/63.2.127
Harada S, Tachikawa H, Kawanishi Y, 2003, A possible association between an insertion/deletion polymorphism of the NQO2 gene and schizophrenia. Psychiatr Genet, 13: 205–209. https://doi.org/10.1097/00041444-200312000-00003
Okada S, Farin FM, Stapleton P, et al., 2005, No associations between Parkinson’s disease and polymorphisms of the quinone oxidoreductase (NQO1, NQO2) genes. Neurosci Lett, 375: 178–180. https://doi.org/10.1016/j.neulet.2004.11.009
Wang W, Jaiswal AK, 2004, Sp3 repression of polymorphic human NRH: quinone oxidoreductase 2 gene promoter. Free Radic Biol Med, 37: 1231–1243. https://doi.org/10.1016/j.freeradbiomed.2004.06.042
Benoit CE, Bastianetto S, Brouillette J, et al., 2010, Loss of quinone reductase 2 function selectively facilitates learning behaviors. J Neurosci, 30: 12690–12700. https://doi.org/10.1523/JNEUROSCI.2808-10.2010
Brouillette J, Quirion R, 2008, Transthyretin: A key gene involved in the maintenance of memory capacities during aging. Neurobiol Aging, 29: 1721–1732. https://doi.org/10.1016/j.neurobiolaging.2007.04.007
Lin R, Liang J, Luo M, 2021, The raphe dopamine system: roles in salience encoding, memory expression, and addiction. Trends Neurosci, 44: 366–377. https://doi.org/10.1016/j.tins.2021.01.002
Voronin MV, Kadnikov IA, Zainullina LF, et al., 2021, Neuroprotective properties of quinone reductase 2 inhibitor M-11, a 2-mercaptobenzimidazole derivative. Int J Mol Sci, 22: 13061. https://doi.org/10.3390/ijms222313061
Umek N, Geršak B, Vintar N, et al., 2018, Dopamine autoxidation is controlled by acidic pH. Front Mol Neurosci, 11: 467. https://doi.org/10.3389/fnmol.2018.00467
Váradi C, 2020, Clinical features of Parkinson’s disease: The evolution of critical symptoms. Biology (Basel), 9: 103. https://doi.org/10.3390/biology9050103
Diederich NJ, Uchihara T, Grillner S, et al., 2020, The Evolution-Driven Signature of Parkinson’s Disease. Trends Neurosci, 43: 475–492. https://doi.org/10.1016/j.tins.2020.05.001
Nobili A, Latagliata EC, Viscomi MT, et al., 2017, Dopamine neuronal loss contributes to memory and reward dysfunction in a model of Alzheimer’s disease. Nat Commun, 8: 14727. https://doi.org/10.1038/ncomms14727
Kaldun JC, Lone SR, Camps AMH, et al., 2021, Dopamine, sleep, and neuronal excitability modulate amyloid-β- mediated forgetting in Drosophila. PLoS Biol, 19: e3001412. https://doi.org/10.1371/journal.pbio.3001412
Segura-Aguilar J, Paris I, Muñoz P, et al., 2014, Protective and toxic roles of dopamine in Parkinson’s disease. J Neurochem, 129: 898–915. https://doi.org/10.1111/jnc.12686
Wakamatsu K, Nakao K, Tanaka H, et al., 2019, The oxidative pathway to dopamine-protein conjugates and their pro-oxidant activities: Implications for the neurodegeneration of Parkinson’s disease. Int J Mol Sci, 20: 2575. https://doi.org/10.3390/ijms20102575
Boutin JA, Thomassin J, Siest G, et al., 1985, Heterogeneity of hepatic microsomal UDP-glucuronosyltransferase activities. Biochem Pharmacol, 34: 2235–2249. https://doi.org/10.1016/0006-2952(85)90777-4
Mackenzie PI, Bock KW, Burchell B, et al., 2005, Nomenclature update for the mammalian UDP glycosyltransferase (UGT) gene superfamily. Pharmacogenet Genomics, 15: 677–685. https://doi.org/10.1097/01.fpc.0000173483.13689.56
Ito T, Katagiri C, Ikeno S, et al., 2001, Phenobarbital following phototherapy for Crigler-Najjar syndrome type II with good fetal outcome: A case report. J Obstet Gynaecol Res, 27: 33–35. https://doi.org/10.1111/j.1447-0756.2001.tb01212.x
Passuello V, Puhl AG, Wirth S, et al., 2009, Pregnancy outcome in maternal Crigler-Najjar syndrome type II: A case report and systematic review of the literature. Fetal Diagn Ther, 26: 121–126. https://doi.org/10.1159/000238122
Chen B, Tong X, Zhang X, et al., 2022, Sulfation modification of dopamine in brain regulates aggregative behavior of animals. Natl Sci Rev, 9: nwab163. https://doi.org/10.1093/nsr/nwab163
Idris M, Mitchell DJ, Gordon R, et al., 2020, Interaction of the brain-selective sulfotransferase SULT4A1 with other cytosolic sulfotransferases: Effects on protein expression and function. Drug Metab Dispos, 48: 337–344. https://doi.org/10.1124/dmd.119.089714
Bock KW, Bock-Hennig BS, 2010, UDP-glucuronosyltransferases (UGTs): From purification of Ah-receptor-inducible UGT1A6 to coordinate regulation of subsets of CYPs, UGTs, and ABC transporters by nuclear receptors. Drug Metab Rev, 42: 6–13. https://doi.org/10.3109/03602530903205492
Yeager RL, Reisman SA, Aleksunes LM, et al., 2009, Introducing the “TCDD-inducible AhR-Nrf2 gene battery”. Toxicol Sci, 111: 238–246. https://doi.org/10.1093/toxsci/kfp115
Rashid MH, Babu D, Siraki AG, 2021, Interactions of the antioxidant enzymes NAD(P)H: Quinone oxidoreductase 1 (NQO1) and NRH: Quinone oxidoreductase 2 (NQO2) with pharmacological agents, endogenous biochemicals and environmental contaminants. Chem Biol Interact, 345: 109574. https://doi.org/10.1016/j.cbi.2021.109574