Selective laser melted Fe–30Mn–6Cu alloy: A multifunctional candidate for MRI-compatible, biodegradable, antibacterial, and biocompatible orthopedic implants
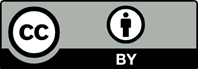
The orthopedic potential of biodegradable iron (Fe)–manganese (Mn)–copper (Cu) alloys remains insufficiently defined, necessitating comprehensive investigation into their mechanical properties, wear resistance, magnetic resonance imaging compatibility, biodegradation behavior, antibacterial efficacy, cytocompatibility, and osteogenic differentiation capacity. This study systematically addresses these aspects through microstructural characterization, mechanical testing, and biological evaluations of Fe–30Mn–6Cu alloy fabricated via selective laser melting (SLM). For comparison, a Cu-free Fe–30Mn alloy was fabricated under similar SLM conditions. The incorporation of 6 wt.% Cu into Fe–30Mn stabilized the γ-austenite phase, enhanced yield strength, improved wear resistance, accelerated electrochemical biodegradation, and imparted strong antibacterial activity. The SLMed Fe–30Mn– 6Cu (i) exhibited a fully γ-austenite microstructure with fine equiaxed grains (~7 μm) containing Cu-enriched intergranular second-phase particles; (ii) demonstrated a yield strength of ~230 MPa—approximately ~24% higher than that of SLMed Fe– 30Mn—along with improved tribological performance, a reduced hysteresis loop area indicating extremely low saturation magnetization and magnetic susceptibility, and a biodegradation rate three times higher compared to the Cu-free counterpart; and (iii) achieved a bacteriostatic rate exceeding 99% against Escherichia coli and Staphylococcus aureus, alongside excellent cytocompatibility and promotion of osteogenic differentiation in MC3T3-E1 cells. These findings provide insights into the structure–property–function relationship of multifunctional Fe–Mn–Cu alloys and their promising applicability in orthopedic implants.

- Liu Z, Zhao MC, Yin D, Zhao YC, Atrens A. Bio-functional niobium-based metallic biomaterials: Exploring their physicomechanical properties, biological significance, and implant applications. Acta Biomater. 2025;192:1-27. doi: 10.1016/j.actbio.2024.12.036
- Zhang Y, Roux C, Rouchaud A, et al. Recent advances in Fe-based bioresorbable stents: materials design and biosafety. Bioact Mater. 2023;31:333-354. doi: 10.1016/j.bioactmat.2023.07.024
- Putra NE, Leeflang MA, Minneboo M, et al. Extrusion-based 3D printed biodegradable porous iron. Acta Biomater. 2021;121:741-756. doi: 10.1016/j.actbio.2020.11.022
- Li Y, Jahr H, Lietaert K, et al. Additively manufactured biodegradable porous iron. Acta Biomater. 2018; 77:380-393. doi: 10.1016/j.actbio.2019.10.034
- Lin W, Qin L, Qi H, et al. Long-term in vivo corrosion behavior, biocompatibility and bioresorption mechanism of a bioresorbable nitrided iron scaffold. Acta Biomater. 2017;54:454-468. doi: 10.1016/j.actbio.2017.03.020
- Zhao Y, Feng J, Yu H, et al. Comparative study on biodegradation of pure iron prepared by microwave sintering and laser melting. Materials (Basel). 2022; 15(4):1604. doi: 10.3390/ma15041604
- Liu B, Zheng YF. Effects of alloying elements (Mn, Co, Al, W, Sn, B, C and S) on biodegradability and in vitro biocompatibility of pure iron. Acta Biomater. 2011;7(3):1407-1420. doi: 10.1016/j.actbio.2010.11.001
- Li S, Ren J, Wang X, et al. Dilemmas and countermeasures of Fe-based biomaterials for next-generation bone implants. J Mater Res Technol. 2022;20:2034-2050. doi: 10.1016/j.jmrt.2022.07.089
- Putra NE, Leeflang MA, Taheri P, et al. Extrusion-based 3D printing of ex situ -alloyed highly biodegradable MRI-friendly porous iron-manganese scaffolds. Acta Biomater. 2021;134:774-790. doi: 10.1016/j.actbio.2021.07.042
- He J, He F-L, Li D-W, et al. Advances in Fe-based biodegradable metallic materials. RSC Adv. 2016;6:112819-112838. doi: 10.1039/c6ra20594a
- Filli L, Luechinger R, Frauenfelder T, et al. Metal-induced artifacts in computed tomography and magnetic resonance imaging: comparison of a biodegradable magnesium alloy versus titanium and stainless steel controls. Skeletal Radiol. 2015;44(6):849-856. doi: 10.1007/s00256-014-2057-5
- Campoccia D, Montanaro L, Arciola CR. The significance of infection related to orthopedic devices and issues of antibiotic resistance. Biomaterials. 2006;27(11):2331-2339. doi: 10.1016/j.biomaterials.2005.11.044
- Guo Y, Zhao M, Xie B, et al. In vitro corrosion resistance and antibacterial performance of novel Fe-xCu biomedical alloys prepared by selective laser melting. Adv Eng Mater. 2021;23:2001000. doi: 10.1002/adem.202001000
- Cordova LA, Stresing V, Gobin B, et al. Orthopaedic implant failure: aseptic implant loosening--the contribution and future challenges of mouse models in translational research. Clin Sci (Lond). 2014;127(5):277-293. doi: 10.1042/CS20130338
- Li X, Zhao YC, Yin D, et al. Microwave-sintered nano- SiC reinforced 8SiC/Ti-3Cu composite: fabrication, wear resistance, antibacterial function, and biocompatibility. Adv Healthc Mater. 2025;14(6):e2403626. doi: 10.1002/adhm.202403626
- Hermawan H, Purnama A, Dube D, Couet J, Mantovani D. Fe-Mn alloys for metallic biodegradable stents: degradation and cell viability studies. Acta Biomater. 2010;6(5):1852-1860. doi: 10.1016/j.actbio.2009.11.025
- Liu P, Huang Q, Shan Q, et al. Achieving exceptional work-hardening capability of additively-manufactured multiphase Fe-Mn alloys via multiple deformation mechanisms, Int J Plast. 2024;173:103871. doi: 10.1016/j.ijplas.2023.103871
- Schinhammer M, Haenzi AC, Loeffler JF, Uggowitzer PJ. Design strategy for biodegradable Fe-based alloys for medical applications. Acta Biomater. 2010;6:1705-1713. doi: 10.1016/j.actbio.2009.07.039
- Li JL, Zhao MC, Zhao YC, et al. Customization and prospects of friction stirprocessing for improving the biomedicalproperties of metallic implants fororthopedic applications. J Mater Res Technol. 2025;34:2133-2149. doi: 10.1016/j.jmrt.2024.12.191
- Mandal S, Viraj, Nandi SK, Roy M. Effects of multiscale porosity and pore interconnectivity on in vitro and in vivo degradation and biocompatibility of Fe–Mn–Cu scaffolds. J Mater Chem B. 2021;9:4340-4354. doi: 10.1039/D1TB00641J
- Zhao Y, Tang Y, Zhao M, et al. Graphene oxide reinforced iron matrix composite with enhanced biodegradation rate prepared by selective laser melting. Adv Eng Mater. 2019;21:1900314. doi: 10.1002/adem.201900314
- Zhao YC, Tang Y, Zhao MC, et al. Study on Fe-xGO composites prepared by selective laser melting: microstructure, hardness, biodegradation and cytocompatibility. JOM 2020;72: 1163-1174 (2020). doi: 10.1007/s11837-019-03814-z
- Zhao, MC., Zhao, YC., Yin, DF. et al. Biodegradation behavior of coated as-extruded Mg–Sr alloy in simulated body fluid. Acta Metall Sin. (Engl Lett.) 2019;32:1195-1206. doi: 10.1007/s40195-019-00892-5
- Cherqaoui A, Cao QN, Gatto ML, Paternoster C, Mengucci P, Mantovani D. Degradation behavior of austenite, ferrite, and martensite present in biodegradable Fe-based alloys in three protein-rich pseudo-physiological solutions. Bioact Mater. 2024;41:96-107. doi: 10.1016/j.bioactmat.2024.06.025
- Dargusch MS, Venezuela J, Dehghan-Manshadi A, et al. In vivo evaluation of bioabsorbable Fe-35Mn-1Ag: first reports on in vivo hydrogen gas evolution in Fe-based implants. Adv Healthc Mater. 2021;10(2):e2000667. doi: 10.1002/adhm.202000667
- Ren G, Huang L, Hu K, et al. Enhanced antibacterial behavior of a novel Cu-bearing high-entropy alloy. J Mater Sci Technol. 2022;117:158-166. doi: 10.1016/j.jmst.2022.02.001
- Yan X, Wan P, Tan L, Zao M, Shuai C, Yang K. Influence of hybrid extrusion and solution treatment on the microstructure and degradation behavior of Mg-0.1Cu alloy. Mater Sci Eng: B. 2018;229:105-117. doi: 10.1016/j.mseb.2017.12.033
- Goudarzi P, Moazami-Goudarzi M, Masoudi A. Sintering, microstructure and properties of absorbable Fe–Mn-xCu alloys. Mater Chem Phys. 2022;287:126368. doi: 10.1016/j.matchemphys.2022.126368
- Zhao Y, Cao Y, Wen W, et al. Effects of Mn content on austenite stability and mechanical properties of low Ni alumina-forming austenitic heat-resistant steel: a first-principles study. Sci Rep. 2023;13(1):5769. doi: 10.1038/s41598-023-32968-9
- Martínez J, Cotes SM, Cabrera AF, Desimoni J, Fernández Guillermet A. On the relative fraction of ε martensite in γ-Fe–Mn alloys. Mater Sci Eng A. 2005;408:26-32. doi: 10.1016/j.msea.2005.06.019
- Dargusch MS, Dehghan-Manshadi A, Shahbazi M, et al. Exploring the role of manganese on the microstructure, mechanical properties, biodegradability, and biocompatibility of porous iron-based scaffolds. ACS Biomater Sci Eng. 2019;5(4):1686-1702. doi: 10.1021/acsbiomaterials.8b01497
- Xie B, Zhao M-C, Tao J-X, et al. Comparison of the biodegradation of ZK30 subjected to solid solution treating and selective laser melting. J Mater Res Technol. 2021;10:722-729. doi: 10.1016/j.jmrt.2020.12.041
- Ji H, Zhao M-C, Xie B, et al. Corrosion and antibacterial performance of novel selective-laser-melted (SLMed) Ti-xCu biomedical alloys. J Alloys Compd. 2021;864:158415. doi: 10.1016/j.jallcom.2020.158415
- Wu S, Liu X, Yeung KWK, Liu C, Yang X. Biomimetic porous scaffolds for bone tissue engineering. Mater Sci Eng Rep. 2014;80:1–36. doi: 10.1016/j.mser.2014.04.001
- Wu M, Zhao M, Cai Y, Yao J, Wang P, Atrens A. Recent advances in bio-functional Ta-based bone materials: materials design and bioactivity. Int J Extrem Manuf. 2024;6:062010. doi: 10.1088/2631-7990/ad7b03
- Ryan G, Pandit A, Apatsidis DP. Fabrication methods of porous metals for use in orthopaedic applications. Biomaterials. 2006;27:2651-2670. doi: 10.1016/j.biomaterials.2005.12.002
- Zhang W, Tan L, Ni D, et al. Effect of grain refinement and crystallographic texture produced by friction stir processing on the biodegradation behavior of a Mg-Nd-Zn alloy. J Mater Sci Technol. 2019;35(5):777-783. doi: 10.1016/j.jmst.2018.11.025
- Song Z, Cai Y, Li X, et al. Fresh insights into structure– function-integrated self-antibacterial Cu-containing Al alloys: giving Al alloys a new function. Mater Horiz. 2025;12:814-832. doi: 10.1039/D4MH00770K
- Wang KX, Yin D, Zhao Y, Atrens A, Zhao M. Microstructural evolution upon heat treatments and its effect on corrosion in Al-Zn-Mg alloys containing Sc and Zr. J Mater Res Technol. 2020;9:5077-5089. doi: 10.1016/j.jallcom.2022.168001
- Liu P, Wu H, Liang L, et al. Microstructure, mechanical properties and corrosion behavior of additively-manufactured Fe–Mn alloys. Mater Sci Eng A. 2022;852:143585. doi: 10.1016/j.msea.2022.143585
- Bhadeshia H, Honeycombe R. Chapter 4 - Solutes that substitute for iron. In: Bhadeshia H, Honeycombe R (eds.). Steels: Microstructure and Properties, Fourth Edition, Butterworth-Heinemann; 2017:101-134. doi: 10.1016/B978-0-08-100270-4.00004-4
- Bairagi D, Mandal S. A comprehensive review on biocompatible Mg-based alloys as temporary orthopaedic implants: current status, challenges, and future prospects. J Magn Alloys. 2022;10:627-669. doi: 10.1016/j.jma.2021.09.005
- Wang X, Xu S, Zhou S, et al. Topological design and additive manufacturing of porous metals for bone scaffolds and orthopaedic implants: a review. Biomaterials. 2016;83:127-141. doi: 10.1016/j.biomaterials.2016.01.012
- Heiden M, Nauman E, Stanciu L. Bioresorbable Fe-Mn and Fe-Mn-HA materials for orthopedic implantation: enhancing degradation through porosity control. Adv Healthc Mater. 2017;6(13):1700120. doi: 10.1002/adhm.201700120
- Hermawan H, Dubé D, Mantovani D. Development of degradable Fe-35Mn alloy for biomedical application. Adv Mater Res. 2006;15–17:107-112. doi: 10.4028/www.scientific.net/AMR.15-17.107
- Mandal S, Kishore AV, Mandal S, et al. Controlled nano Cu precipitation through age treatment: a method to enhance the biodegradation, mechanical, antimicrobial properties and biocompatibility of Fe-20Mn-3Cu alloys. Acta Biomater. 2023;168:650-669. doi: 10.1016/j.actbio.2023.07.004
- Lu X, Zhang D, Xu W, et al. The effect of Cu content on corrosion, wear and tribocorrosion resistance of Ti-Mo- Cu alloy for load-bearing bone implants. Corros Sci. 2020;177:109007. doi: 10.1016/j.corsci.2020.109007
- Lintzen S, von Appen J, Hallstedt B, Dronskowski R. The Fe–Mn enthalpy phase diagram from first principles. J Alloys Compd. 2013;577:370-375. doi: 10.1016/j.jallcom.2013.06.006
- Jurgeleit T, Quandt E, Zamponi C. Magnetron sputtering as a fabrication method for a biodegradable Fe32Mn alloy. Materials (Basel). 2017;10(10):1196. doi: 10.3390/ma10101196
- Schenck JF. The role of magnetic susceptibility in magnetic resonance imaging: MRI magnetic compatibility of the first and second kinds. Med Phys. 1996;23:815-850. doi: 10.1118/1.597854
- Lee MK, Lee H, Park C, et al. Accelerated biodegradation of iron-based implants via tantalum-implanted surface nanostructures. Bioact Mater. 2021;9:239-250. doi: 10.1016/j.bioactmat.2021.07.003
- Wang P, Wang Q, Wu D, et al. Enhancing osteogenic bioactivities of coaxial electrospinning nano-scaffolds through incorporating iron oxide nanoparticles and icaritin for bone regeneration. Nano Res. 2024;17:6430-6442. doi: 10.1007/s12274-024-6656-8
- Gao YN, Yang HT, Qiu ZF, et al. Long-term efficacy, safety and biocompatibility of a novel sirolimus eluting iron bioresorbable scaffold in a porcine model. Bioact Mater. 2024;39:135-146. doi: 10.1016/j.bioactmat.2024.05.027
- Liu B, Zheng YF, Ruan L. In vitro investigation of Fe30Mn6Si shape memory alloy as a potential biodegradable metallic material. Mater Lett. 2011; 65:540-543. doi: 10.1016/j.matlet.2010.10.068
- Zhang W, Zao MC, Wang Z, et al. Enhanced initial biodegradation resistance of the biomedical Mg-Cu alloy by surface nanomodification. J Magnes Alloys. 2023;11:2776-2788. doi: 10.1016/j.jma.2021.12.013
- Venezuela J, Dargusch MS. Addressing the slow corrosion rate of biodegradable Fe-Mn: current approaches and future trends. Curr Opin Solid State Mater Sci. 2020; 24:100822. doi: 10.1016/j.cossms.2020.100822
- Godoy-Gallardo M, Eckhard U, Delgado LM, et al. Antibacterial approaches in tissue engineering using metal ions and nanoparticles: from mechanisms to applications. Bioact Mater. 2021;6:4470-4490. doi: 10.1016/j.bioactmat.2021.04.033
- Zhang E, Zao X, Hu J, Wang R, Fu S, Qin G. Antibacterial metals and alloys for potential biomedical implants. Bioact Mater. 2021;6:2569-2612. doi: 10.1016/j.bioactmat.2021.01.033
- Nan L, Liu Y, Lü M, Yang K. Study on antibacterial mechanism of copper-bearing austenitic antibacterial stainless steel by atomic force microscopy. J Mater Sci Matern Med. 2008;19:3057-3062. doi: 10.1016/j.bioactmat.2021.01.030
- Zheng YF, Gu XN, Witte F. Biodegradable metals. Mater Sci Eng R: Rep. 2014;77:1–34. doi: 10.1016/j.mser.2014.01.001
- Yuan Y, Jin S, Qi X, et al. Osteogenesis stimulation by copper-containing 316L stainless steel via activation of akt cell signaling pathway and Runx2 upregulation. J Mater Sci Technol. 2019;35:2727-2733. doi: 10.1016/j.jmst.2019.04.028