Biofabrication of tri-layered nerve guide conduits for peripheral nerve regeneration: Synergizing melt-electrowriting of polymeric fibers and extrusion-based 3D bioprinting
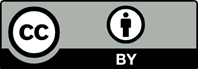
Nerve guide conduits (NGCs) are promising alternatives to autografts for the treatment of peripheral nerve injuries. These conduits are designed to replace the nerve tissue that has been damaged or removed from the injured nerve region. An ideal nerve conduit should effectively bridge the nerve gap and induce nerve cell growth and regeneration. Current FDA-approved NGCs predominantly address gaps smaller than 2 cm and are fabricated from rigid synthetic polymers. Nevertheless, cells prefer softer substrates that more closely mimic the extracellular matrix (ECM). While hydrogels emerge as an ideal ECM-mimicking material, their application is limited by challenges in suturing, maintaining structural integrity, and susceptibility to rapid biodegradation. In this study, we propose a tri-layered NGC biofabricated by combining melt-electrowriting (MEW) and extrusion-based bioprinting, thereby facilitating three functionalities—the outer layer of MEW-polycaprolactone (PCL) fiber monophasic structure for mechanical integrity, the middle layer of MEW-PCL aligned fibers providing topographical cues for axonal directionality, and the inner bioprinted gelatin methacryloyl layer for encapsulating cells in an ECM-mimicking matrix (~7 kPa stiffness matching nerve tissues). In addition to having tunable mechanical properties (by changing the outer layer design), these biocompatible materials are cost-effective, easily biofabricated, highly tunable for drug-loading, and can support the growth, proliferation, and differentiation of human neural stem cells to peripheral neurons, making the proposed tri-layered NGCs promising candidates for treating long nerve gap injuries.

- Kuffler DP, Foy C. Restoration of neurological function following peripheral nerve trauma. Int J Mol Sci. 2020;21(5):1808. doi: 10.3390/ijms21051808
- Qiao F, Jeongsim H, Shuxin L. Nonsteroidal anti-inflammatory drugs promote axon regeneration via RhoA inhibition. J Neurosci. 2007;27(15):4154. doi: 10.1523/JNEUROSCI.4353-06.2007
- Maugeri G, D’Agata V, Trovato B, et al. The role of exercise on peripheral nerve regeneration: from animal model to clinical application. Heliyon. 2021;7(11):e08281. doi: 10.1016/j.heliyon.2021.e08281
- Kornfeld T, Vogt PM, Radtke C. Nerve grafting for peripheral nerve injuries with extended defect sizes. Wien Med Wochenschr. 2019;169(9):240-251. doi: 10.1007/s10354-018-0675-6
- Soman SS, Vijayavenkataraman S. Perspectives on 3D bioprinting of peripheral nerve conduits. Int J Mol Sci. 2020;21(16):5792. doi: 10.3390/ijms21165792
- Gao Y, Wang Y-l, Kong D, et al. Nerve autografts and tissue-engineered materials for the repair of peripheral nerve injuries: a 5-year bibliometric analysis. Neural Regen Res. 2015;10(6):1003-1008. doi: 10.4103/1673-5374.158369
- Ouyang L, Armstrong James PK, Lin Y, et al. Expanding and optimizing 3D bioprinting capabilities using complementary network bioinks. Sci Adv. 2020;6(38):eabc5529. doi: 10.1126/sciadv.abc5529
- Elkhoury K, Morsink M, Tahri Y, et al. Synthesis and characterization of C2C12-laden gelatin methacryloyl (GelMA) from marine and mammalian sources. Int J Biol Macromol. 2021;183:918-926. doi: 10.1016/j.ijbiomac.2021.05.040
- Soman S, Govindraj M, Al Hashimi N. Bioprinting of human neural tissues using a sustainable marine tunicate-derived bioink for translational medicine applications Int J Bioprint. 2022;8(4):604. doi: 10.18063/ijb.v8i4.604
- Govindharaj M, Al Hashemi NS, Soman SS, Vijayavenkataraman S. Bioprinting of bioactive tissue scaffolds from ecologically-destructive fouling tunicates. J Clean Prod. 2022;330:129923. doi: 10.1016/j.jclepro.2021.129923
- Elkhoury K, Morsink M, Sanchez-Gonzalez L, Kahn C, Tamayol A, Arab-Tehrany E. Biofabrication of natural hydrogels for cardiac, neural, and bone tissue engineering applications. Bioact Mater. 2021;6(11):3904-3923. doi: 10.1016/j.bioactmat.2021.03.040
- Van Den Bulcke AI, Bogdanov B, De Rooze N, Schacht EH, Cornelissen M, Berghmans H. Structural and rheological properties of methacrylamide modified gelatin hydrogels. Biomacromolecules. 2000;1(1):31-38. doi: 10.1021/bm990017d
- Chen Z, Wang L, Chen C, et al. NSC-derived extracellular matrix-modified GelMA hydrogel fibrous scaffolds for spinal cord injury repair. NPG Asia Mater. 2022;14(1):20. doi: 10.1038/s41427-022-00368-6
- Kim J, Raja N, Choi YJ, et al. Enhancement of properties of a cell-laden GelMA hydrogel-based bioink via calcium phosphate phase transition. Biofabrication. 2024;16(1):015010. doi: 10.1088/1758-5090/ad05e2
- Yue K, Trujillo-de Santiago G, Alvarez MM, Tamayol A, Annabi N, Khademhosseini A. Synthesis, properties, and biomedical applications of gelatin methacryloyl (GelMA) hydrogels. Biomaterials. 2015;73:254-271. doi: 10.1016/j.biomaterials.2015.08.045
- Xu W, Molino BZ, Cheng F, et al. On low-concentration inks formulated by nanocellulose assisted with gelatin methacrylate (GelMA) for 3D printing toward wound healing application. ACS Appl Mater Interfaces. 2019;11(9):8838-8848. doi: 10.1021/acsami.8b21268
- Petcu EB, Midha R, McColl E, Popa-Wagner A, Chirila TV, Dalton PD. 3D printing strategies for peripheral nerve regeneration. Biofabrication. 2018;10(3):032001. doi: 10.1088/1758-5090/aaaf50
- Jin Z, Li Y, Yu K, et al. 3D printing of physical organ models: recent developments and challenges. Adv Sci. 2021;8(17):2101394. doi: 10.1002/advs.202101394
- O’Neill KL, Dalton PD. A decade of melt electrowriting. Small Methods. 2023;7(7):e2201589. doi: 10.1002/smtd.202201589
- Kim J, Bakirci E, O’Neill KL, Hrynevich A, Dalton PD. Fiber bridging during melt electrowriting of poly(ε-Caprolactone) and the influence of fiber diameter and wall height. Macromol Mater Eng. 2021;306(3):2000685. doi: 10.1002/mame.202000685
- Weekes A, Wehr G, Pinto N, et al. Highly compliant biomimetic scaffolds for small diameter tissue-engineered vascular grafts (TEVGs) produced via melt electrowriting (MEW). Biofabrication. 2023;16(1):015017. doi: 10.1088/1758-5090/ad0ee1
- Keßler L, Mirzaei Z, Kade JC, Luxenhofer R. Highly porous and drug-loaded amorphous solid dispersion microfiber scaffolds of indomethacin prepared by melt electrowriting. ACS Appl Polymer Mater. 2023;5(1):913-922. doi: 10.1021/acsapm.2c01845
- Akhtar R, Sherratt MJ, Cruickshank JK, Derby B. Characterizing the elastic properties of tissues. Mater Today. 2011;14(3):96-105. doi: 10.1016/S1369-7021(11)70059-1
- Caliari SR, Burdick JA. A practical guide to hydrogels for cell culture. Nat Methods. 2016;13(5):405-414. doi: 10.1038/nmeth.3839
- Takeya H, Itai S, Kimura H, et al. Schwann cell-encapsulated chitosan-collagen hydrogel nerve conduit promotes peripheral nerve regeneration in rodent sciatic nerve defect models. Sci Rep. 2023;13(1):11932. doi: 10.1038/s41598-023-39141-2
- Lee HS, Jeon EY, Nam JJ, et al. Development of a regenerative porous PLCL nerve guidance conduit with swellable hydrogel-based microgrooved surface pattern via 3D printing. Acta Biomater. 2022;141:219-232. doi: 10.1016/j.actbio.2022.01.042
- Fadia NB, Bliley JM, DiBernardo GA, et al. Long-gap peripheral nerve repair through sustained release of a neurotrophic factor in nonhuman primates. Sci Transl Med. 2020;12(527):eaav7753. doi: 10.1126/scitranslmed.aav7753
- Elkhoury K, Sanchez-Gonzalez L, Lavrador P, et al. Gelatin methacryloyl (GelMA) nanocomposite hydrogels embedding bioactive naringin liposomes. Polymers. 2020;12(12):2944. doi: 10.3390/polym12122944
- Chen T, Xia Y, Zhang L, et al. Loading neural stem cells on hydrogel scaffold improves cell retention rate and promotes functional recovery in traumatic brain injury. Mater Today Bio. 2023;19:100606. doi: 10.1016/j.mtbio.2023.100606
- Vijayavenkataraman S, Kannan S, Cao T, Fuh JYH, Sriram G, Lu WF. 3D-printed PCL/PPy conductive scaffolds as three-dimensional porous nerve guide conduits (NGCs) for peripheral nerve injury repair. Front Bioeng Biotechnol. 2019;7:266. doi: 10.3389/fbioe.2019.00266
- Frost HK, Andersson T, Johansson S, et al. Electrospun nerve guide conduits have the potential to bridge peripheral nerve injuries in vivo. Sci Rep. 2018;8(1):16716. doi: 10.1038/s41598-018-34699-8
- Gao S, Tang Y, Sun W, et al. 3D-bioprinted GelMA nerve guidance conduits promoted peripheral nerve regeneration by inducing trans-differentiation of MSCs into SCLCs via PIEZO1/YAP axis. Mater Today Adv. 2023;17:100325. doi: 10.1016/j.mtadv.2022.100325
- Borschel GH, Kia KF, Kuzon WM, Dennis RG. Mechanical properties of acellular peripheral nerve. J Surg Res. 2003;114(2):133-139. doi: 10.1016/S0022-4804(03)00255-5
- Ju M-S, Lin C-CK, Fan J-L, Chen R-J. Transverse elasticity and blood perfusion of sciatic nerves under in situ circular compression. J Biomech. 2006;39(1):97-102. doi: 10.1016/j.jbiomech.2004.10.026
- Xiao S, Zhao T, Wang J, et al. Gelatin methacrylate (GelMA)-based hydrogels for cell transplantation: an effective strategy for tissue engineering. Stem Cell Rev Rep. 2019;15(5):664-679. doi: 10.1007/s12015-019-09893-4
- Shahidi S, Janmaleki M, Riaz S, Sanati Nezhad A, Syed N. A tuned gelatin methacryloyl (GelMA) hydrogel facilitates myelination of dorsal root ganglia neurons in vitro. Mater Sci Eng C. 2021;126:112131. doi: 10.1016/j.msec.2021.112131
- Ye W, Li H, Yu K, et al. 3D printing of gelatin methacrylate-based nerve guidance conduits with multiple channels. Mater Design. 2020;192:108757. doi: 10.1016/j.matdes.2020.108757
- O’Connell CD, Zhang B, Onofrillo C, et al. Tailoring the mechanical properties of gelatin methacryloyl hydrogels through manipulation of the photocrosslinking conditions. Soft Matter. 2018;14(11):2142-2151. doi: 10.1039/C7SM02187A
- Annabi N, Nichol JW, Zhong X, et al. Controlling the porosity and microarchitecture of hydrogels for tissue engineering. Tissue Eng Part B Rev. 2010;16(4):371-383. doi: 10.1089/ten.teb.2009.0639
- Murphy CM, O’Brien FJ. Understanding the effect of mean pore size on cell activity in collagen-glycosaminoglycan scaffolds. Cell Adhes Migr. 2010;4(3):377-381. doi: 10.4161/cam.4.3.11747
- Yin H, Zhu M, Wang Y, Luo L, Ye Q, Lee BH. Physical properties and cellular responses of gelatin methacryloyl bulk hydrogels and highly ordered porous hydrogels. Front Soft Matter. 2023;2;1101680. doi: 10.3389/frsfm.2022.1101680
- Gu L, Li T, Song X, et al. Preparation and characterization of methacrylated gelatin/bacterial cellulose composite hydrogels for cartilage tissue engineering. Regen Biomater. 2020;7(2):195-202. doi: 10.1093/rb/rbz050
- Yin B, Yang H, Yang M. Integrating soft hydrogel with nanostructures reinforces stem cell adhesion and differentiation. J Compos Sci. 2022;6(1):19. doi: 10.3390/jcs6010019
- Merivaara A, Koivunotko E, Manninen K, et al. Stiffness-controlled hydrogels for 3D cell culture models. Polymers. 2022;14(24):5530. doi: 10.3390/polym14245530
- Fan L, Liu C, Chen X, et al. Directing induced pluripotent stem cell derived neural stem cell fate with a three-dimensional biomimetic hydrogel for spinal cord injury repair. ACS Appl Mater Interfaces. 2018;10(21):17742-17755. doi: 10.1021/acsami.8b05293
- Mijailovic AS, Galarza S, Raayai-Ardakani S, et al. Localized characterization of brain tissue mechanical properties by needle induced cavitation rheology and volume controlled cavity expansion. J Mech Behav Biomed Mater. 2021;114:104168. doi: 10.1016/j.jmbbm.2020.104168
- Weltman A, Yoo J, Meng E. Flexible, penetrating brain probes enabled by advances in polymer microfabrication. Micromachines (Basel). 2016;7(10):180. doi: 10.3390/mi7100180
- Wu W, Dong Y, Liu H, et al. 3D printed elastic hydrogel conduits with 7,8-dihydroxyflavone release for peripheral nerve repair. Materials Today Bio. 2023;20:100652. doi: 10.1016/j.mtbio.2023.100652
- Li A, Hokugo A, Yalom A, et al. A bioengineered peripheral nerve construct using aligned peptide amphiphile nanofibers. Biomaterials. 2014;35(31):8780-8790. doi: 10.1016/j.biomaterials.2014.06.049
- Kim Y-t, Haftel VK, Kumar S, Bellamkonda RV. The role of aligned polymer fiber-based constructs in the bridging of long peripheral nerve gaps. Biomaterials. 2008;29(21):3117-3127. doi: 10.1016/j.biomaterials.2008.03.042
- Hawkins AM, Milbrandt TA, Puleo DA, Hilt JZ. Synthesis and analysis of degradation, mechanical and toxicity properties of poly(β-amino ester) degradable hydrogels. Acta Biomater. 2011;7(5):1956-1964. doi: 10.1016/j.actbio.2011.01.024
- Sarker M, Naghieh S, McInnes AD, Schreyer DJ, Chen X. Strategic design and fabrication of nerve guidance conduits for peripheral nerve regeneration. Biotechnol J. 2018;13(7):e1700635. doi: 10.1002/biot.201700635
- Carvalho CR, Oliveira JM, Reis RL. Modern trends for peripheral nerve repair and regeneration: beyond the hollow nerve guidance conduit. Front Bioeng Biotechnol. 2019;7:337. doi: 10.3389/fbioe.2019.00337
- Zhang K, Zheng H, Liang S, Gao C. Aligned PLLA nanofibrous scaffolds coated with graphene oxide for promoting neural cell growth. Acta Biomater. 2016;37:131-142. doi: 10.1016/j.actbio.2016.04.008
- Yang S, Zhu J, Lu C, et al. Aligned fibrin/functionalized self-assembling peptide interpenetrating nanofiber hydrogel presenting multi-cues promotes peripheral nerve functional recovery. Bioact Mater. 2022;8:529-544. doi: 10.1016/j.bioactmat.2021.05.056
- Puhl DL, Funnell JL, Nelson DW, Gottipati MK, Gilbert RJ. Electrospun fiber scaffolds for engineering glial cell behavior to promote neural regeneration. Bioengineering. 2020;8(1):4. doi: 10.3390/bioengineering8010004
- Zhao Y, Liang Y, Ding S, Zhang K, Mao H-q, Yang Y. Application of conductive PPy/SF composite scaffold and electrical stimulation for neural tissue engineering. Biomaterials. 2020;255:120164. doi: 10.1016/j.biomaterials.2020.120164
- Knight E, Przyborski S. Advances in 3D cell culture technologies enabling tissue-like structures to be created in vitro. J Anat. 2015;227(6):746-756. doi: 10.1111/joa.12257
- Wenzhuo F, Ming Y, Liyang W, et al. Hydrogels for 3D bioprinting in tissue engineering and regenerative medicine: Current progress and challenges. IJB. 2023;9(5):759. doi: 10.18063/ijb.759
- Naganuma T. The relationship between cell adhesion force activation on nano/micro-topographical surfaces and temporal dependence of cell morphology. Nanoscale. 2017;9(35):13171-13186. doi: 10.1039/c7nr04785a
- Sater AP, Rael LT, Tanner AH, et al. Cell death after traumatic brain injury: detrimental role of anoikis in healing. Clin Chim Acta. 2018;482:149-154. doi: 10.1016/j.cca.2018.04.008
- Taddei ML, Giannoni E, Fiaschi T, Chiarugi P. Anoikis: an emerging hallmark in health and diseases. J Pathol. 2011;226(2):380-393. doi: 10.1002/path.3000
- Kanemura Y, Mori H, Kobayashi S, et al. Evaluation of in vitro proliferative activity of human fetal neural stem/ progenitor cells using indirect measurements of viable cells based on cellular metabolic activity. J Neurosci Res. 2002;69(6):869-879. doi: 10.1002/jnr.10377
- An J, Chen B, Tian D, Guo Y, Yan Y, Yang H. Regulation of neurogenesis and neuronal differentiation by natural compounds. Curr Stem Cell Res Ther. 2022;17(8):756-771. doi: 10.2174/1574888X16666210907141447