Microstructure control during cryogenic 3D printing to obtain biomimetic porous and tough cross-scale mineralized collagen bone scaffold
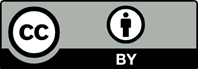
Tissue engineering (TE) is a promising strategy to repair large bone defects by inducing endogenous bone regeneration. The ideal bone TE scaffold should possess high porosity (90%), suitable stiffness (1 MPa), and most importantly, a composition that mimics natural bone, including the same components (mineralized collagen) and cross-macro- and microscale structures. However, existing 3D-printed mineralized collagen bone TE scaffold hardly reproduces the cross-scale structure of natural bone, leading to low porosity (60%) and poor stiffness (100 kPa). To address this challenge, this study applied cryogenic 3D printing, also known as low-temperature field-assisted direct ink writing, to achieve 3D mineralized collagen scaffolds with cross-macro- and microscale structures. The inclusion of numerous micro-pores within the extruded fibers resulted in a porosity of 95%. In addition, through the control of scaffold microstructure and in situ mineralization, Young’s modulus of the cryogenic-printed collagen scaffold can be increased by 240% while maintaining the porosity at 95%, matching the properties of an ideal bone TE scaffold. In summary, this work provides new guidelines for technological innovation and application of cryogenic 3D printing, achieving a biomimetic mineralized collagen bone TE scaffold. In addition, the high porosity of the scaffolds produced by this technology enables these scaffolds to be used in various fields, including impact resistance, wave absorption, thermal insulation, flexible materials, and piezoelectric ceramics, among others.

1. Introduction
Large bone defects caused by traffic accidents and sports injuries present a significant clinical challenge. Currently, surgical treatment strategies, such as autologous/allogeneic grafts and arthroplasty with permanent metal implants, have been used for bone defect repair. However, several disadvantages hinder the broad application of these treatment strategies, such as secondary trauma, resource shortage of autografts/allografts, low cell survival, and the poor degradation rate of the metal graft. To address these challenges, tissue engineering (TE) scaffolds present a promising alternative and have garnered increasing attention recently due to the prospect of inducing endogenous bone regeneration to repair bone defects.1,2
Ideal bone TE scaffolds should possess high porosity (>90%) and suitable stiffness to provide a desirable environment and stimulation cues for osteoblast growth, osteogenesis, and bone regeneration.3,4 An excellent design strategy is to mimic the structure of highly porous and tough natural cancellous bone. Natural bones are highly porous and tough due to their cross-macro- and microscale structure.5,6 In natural bone, the basic bone unit is arranged in a concentric circle, with a lamellar pattern and an interval distance of 1 μm at the microscale.7 In contrast, the bone units are arranged into cortical and cancellous bone at the macroscale. The cross-scale structure (cm– mm–μm) confers cancellous bone a high porosity (>90%), high specific surface area, and more importantly, adequate mechanical strength. A high porosity is important for bone TE scaffold, as the microchannels facilitate nutrient exchange, playing a vital role in sustaining bone structure. Stiffness (durotaxis) is another key aspect of the extracellular matrix (ECM), as it defines compression resistance and affects cell behavior.8 Despite the difference in mechanical strength between these scaffolds and that of natural cancellous bone, it was demonstrated that scaffolds (compression strength: 0.5 kPa–2.7 MPa) can affect cell growth and behavior scaffolds; additionally, ECM with stiffness 1–1.4 MPa was reported to be most desirable for osteogenesis.9–12 However, existing bone TE scaffolds rarely possess both high porosity (>90%) and suitable stiffness (1 MPa Young’s modulus).
Mineralized collagen is the main component of natural bone, and it has been fabricated into composite particles,13 hydrogels,14 films,15 and 3D scaffolds for bone regeneration. In intrafibrillar or interfibrillar collagen mineralization, the nucleation of calcium phosphate crystals results from heterogeneous reactions between collagen and mineral ions. Prenucleation clusters of initial calcium phosphate are proposed to appear as a consequence of homogeneous reactions between the ions alone, and these clusters may also promote crystal formation in additional heterogeneous events with collagen or collagen-bound molecules. Together, these physicochemical events occurring within, on the surface of, and beyond collagen produce a collagen–mineral composite that fully occupies and calcifies both the collagen and the tissue spaces between the collagen fibers,16–22 such as the direct casting of a collagen–hydroxyapatite (HA) mixture.23 The advantage of freeze-casting is the feasible adjustment of collagen and HA ratio; however, the disadvantage of it is the poor control of scaffold shape. Extrusion 3D printing, or direct ink writing, has been applied in collagen bone TE scaffold fabrication due to the high degree of freedom.24 However, the fabrication precision is merely 0.2 mm due to the large nozzle size, which cannot fabricate cross-scale structures at the microscale. For example, HA-collagen scaffolds were fabricated through direct writing or extrusion 3D printing, typically with a fiber diameter of 0.2 mm and a low porosity (<60%).25 To fabricate bone TE scaffolds with a cross-scale structure, cryogenic 3D printing, also known as low-temperature-field-assisted direct writing, is adopted.26 By conducting slurry extrusion in a low-temperature field, the solvent (usually water) will freeze during the deposition process, leading to phase separation of the solvent and solute Upon freeze-drying, the ice crystals are removed, forming micropores (1 μm) inside the extruded fiber (0.1 mm) of the bone TE scaffold (1 cm).27 As a result, a cross-scale scaffold (cm–mm–μm) with high porosity can be created.
Currently, there is limited research focusing on the mechanism of cryogenic 3D printing for the modulation of microstructural and mechanical properties.28 This study utilized a low-temperature field and varying print speeds to investigate the relationship between microstructural and mechanical properties, achieving tough collagen scaffolds with a controlled micro-lamellar pattern. In addition, in situ mineralization was adopted for posttreatment of the mineral collagen composite scaffold. By regulating the lamellar pattern direction and integrating in situ mineralization, the cryogenic 3D-printed scaffold reported a fourfold increase in Young’s modulus and a porosity of over 90%, meeting the porosity and stiffness requirement for bone TE scaffolds. This work offers new guidelines for technological innovation and applications of cryogenic 3D printing technology. Figure 1a displays the cross-scale structure of natural bone. Figure 1b displays the fabrication process of cryogenic 3D printing. The cryogenic 3D printed scaffold has a high porosity and small density.
Figure 1. The cross-scale structure of natural bone and cryogenic 3D-printed artificial bone. (a) The cross-scale (cm–mm–μm) structure of natural bone. Adapted from ref.5 (b) The cryogenic 3D printing process to obtain micro-lamellar structures. (c) The cross-scale (cm–mm–μm) structure of cryogenic 3D-printed artificial bone.
2. Materials and methods
2.1. Collagen slurry preparation
For slurry preparation, 0.3 g decellularized small intestinal submucosa (dSIS) powder (Guangzhou Origin Life Science & Technology Co., Ltd., China) and 0.03 g pepsin (Aladdin, China) were mixed in 10 mL 0.01 M HCl (Chron Chemicals Co., Ltd., China) with constant stirring for 24 h at 35 °C. The dSIS is a decellularized ECM, primarily comprising collagen (>70 wt.%). The obtained slurry was centrifuged for 2 min at 2000 rad/min (Lichen, China) to remove residual fragments and impurities.
2.2. Cryogenic 3D printing
A robust model of the scaffold was constructed using UG12.0 software (Siemens, German). The print path was generated with a filament spacing of 0.6 mm and layer height of 0.25 mm; the slurry was then transferred into a syringe with an inner needle diameter of 0.3 mm. The printing process was then initiated. The moving speeds of the platform (Shanghai Fengbao, China) were set at 3 and 20 mm/s; the air pressure was set at 0.4 and 0.65 MPa, respectively. The overall size of the scaffold was 12 × 12 × 3 mm3. An in-house cryogenic 3D printer was used to print the artificial collagen bones. The scaffold was printed in cubic shape to investigate the microstructure control mechanism. An automatic cooling pump (DLSB-10/80, Zhengzhou Teer Equipment Co., Ltd., China), with a range of –80–99 ± 0.5°C, was used to control the temperature of the cryogenic stage. As the scaffolds formed on the freezing platform, the water contained within the extruded slurry undergoes a phase transition, transforming into ice that penetrates the collagen fibers, thereby establishing a template. Following the subsequent sublimation of the ice template during freeze-drying (Ningbo Scientz Biotechnology Co., Ltd., China), micropores were formed. The orientation of the ice template was meticulously controlled to ensure precise micropore orientation.
2.3. Low-temperature field simulation
The thermal behavior of the cryogenic printing process was modeled using a finite element (FE) model. The initial thermal conditions were captured using an infrared camera (TiX875, Fluke, USA) to calibrate for temperature measurements.
The thermal behavior during cryogenic printing follows Equation (I):
where T (x, y, z) is the thermal field variable, u (ux, uy, uz) is the velocity field, q (qx, qy, qz) is the heat flux vector field, Q is the heat source (J), ρ is the density (kg/m3), Cp is the specific heat capacity (J/kg⋅K), and k is the thermal conductivity (W/m⋅K).
To enhance the consistency between the simulated process and the actual printing process, the geometry, material properties, boundary conditions, and initial conditions were optimized accordingly. Considering the computational capability of the hardware, a 1 mm length printed track in the middle layer of the structure was chosen for simulation (Figure 2a).
Figure 2. Simulation model. (a) Geometry of the printed track: isometric view (left); side view (right); the inset image in (a) shows the location of the simulation model in the whole scaffold. (b) Boundary conditions. (c) Mesh design of the printed track.
2.3.1. Geometry
During the calibration of the cryogenic printing process, an approximate circle geometry was selected as the crosssectional area of the printed track for the newly printed layer (Figure 2a). In order to simplify the calculations, the whole calculations about the low-temperature field in the following research calculations, the FE computational model was used as the contact position of the scaffolds. The choose of FE model is the first step before all calculations.
2.3.2. Material properties
During material preparation for cryogenic printing, the materials were mixed with pure water at 3 wt.%. Due to the low concentration of the mixture and the difficulty in calibrating the physical properties of the target material, pure water was selected as the simulated domain.
2.3.3. Boundary and initial conditions
The simulated process represents the middle layer of the entire cryogenic printing process, where the existing layer has already been printed. Therefore, the connection area of the target printed track and the previously printed tracks was selected as the boundary condition interface, with a temperature of 253 K assigned to this area (Figure 2b). The temperature-dependent physical properties were activated along the printing direction at the speed of the actual printing.
To improve simulation accuracy, an adaptive mesh was designed in the simulation domain, where the quad elements were set at the cross-section of the printed track and swept along the printing direction (Figure 2c). The number of the boundary elements was 7892, while the number of the entire elements was 100,215. The proposed FE model was computed using COMSOL Multiphysics software (COMSOL AB, Sweden).
2.4. In situ mineralization as post-treatment
After printing, in situ mineralization was applied to the 3D collagen scaffolds as post-treatment to improve the mechanical properties of the scaffolds with minimal deformation. The scaffolds were immersion in 95% ethanol containing 1 wt.% 1-ethyl-3-(3-dimethylaminopropyl) carbodiimide (EDC) (Solarbio, China) for 4 h at room temperature. Residual HCl in the collagen was neutralized by incubating the scaffold in 3 wt.% NaHCO3 (Aladdin, China) for 5 min. The obtained scaffolds were washed three times with sterilized distilled water and freezedried for 24 h. The scaffolds underwent a 5-h immersion in mineralization solution A (100 mM sodium carbonate [Macklin, China]; 30 mM sodium chloride [Sinopharm Chemical Reagent Co., Ltd., China]) to facilitate complete absorption of the scaffolds. After that, the scaffolds were transferred to mineralization solution B (100 mM calcium chloride [Sinopharm Chemical Reagent Co., Ltd., China]; 100 mM 4-(2-hydroxyethyl)-1-piperazineethanesulfonic acid [HEPES; Sigma-Aldrich, USA]; 160 mg/mL polyacrylic acid [PAA; Macklin, China]) for 10 h. HEPES buffer was used to maintain the pH of the mineralization solution in a weakly alkaline solution; PAA (30 wt.%) was used to maintain the calcium carbonate in a precursor state and embed the formed precursors inside the scaffold fibers.
2.5. Porosity calculation
Pre-weighed (Wdry) samples with known volume (V) were immersed in water for 1 h to saturate the pores; the samples were then re-weighed (Wsat). Porosity was determined using Equation (II):
where ρ is the density of water. Five samples were tested for each group.
2.6. Characterization of cryogenic 3D-printed scaffolds
The microstructure of the 3D-printed collagen scaffold was observed through a scanning electron microscope, and then elemental analysis was performed using Energy Dispersive Spectrometry (SEM; EDS; Gemini SEM, Zeiss, Germany). The inner microstructures of the printed scaffolds were observed through an X-ray microscope (XRM; Xradia 610 Versa, Zeiss, Germany). Further, the macro-morphology of the collagen scaffold was determined using an optical electron microscope (B011, Supereyes, China). The 3D-printed collagen scaffolds were evaluated for various structural, physicochemical, and mechanical properties. Fourier transform infrared (FTIR) spectroscopy was carried out using Vertex-70 (Bruker, Germany), over a spectral range of 4000–450 cm−1 at a resolution of 0.25 cm−1, using a Deuterated Triglycine Sulfate detector. To verify the in situ mineralized Ca salt in collagen scaffold, thermogravimetric analysis (TGA; STA 6000, PerkinElmer, United States of America [USA]) of the mineralized scaffolds was conducted at a ramp rate of 20 °C/min at a temperature range of 30–1000 °C. The cryogenic 3D-printed collagen scaffolds were subjected to mechanical property testing. A uniaxial compression test was conducted using an electronic universal testing machine (WDW, Harbin Kexin Instrument Co., Ltd., China) under a compression speed of 0.5 mm/min at room temperature to evaluate the Young’s modulus of scaffolds in both dry and mineralized states, with each group comprising three samples. The tensile modulus of the scaffolds in the dry state was measured using the same machine at a tensile rate of 1 mm/min (Figure S1, Supporting Information). The stress–strain curves of the scaffolds were recorded, and Young’s modulus was calculated from the linear slope obtained using Origin software.
2.7. Falling-ball impact experiment
The falling-ball impact test was conducted to examine the mechanical properties of both unmineralized and mineralized samples under dynamic impact conditions. Briefly, a steel ball (50 g) was dropped from a height (h0) of 0.3, 0.5, and 0.7 m onto the samples, and the rebound height (h1) was measured after the experiment. The energy was calculated using Equation (III):
where ΔE represents the change in energy, which is the difference between the initial energy (E₀) at height h₀ and the final energy (E₁) at height h₁. The mass of the ball is denoted by m, and g is the acceleration due to gravity, which is approximately 9.81 m/s² on Earth. The initial and final heights of the ball above a reference point are represented by h₀ and h₁, respectively. This formula shows that the change in gravitational potential energy is directly proportional to the mass of the ball, the acceleration due to gravity, and the difference in height.
2.8. Accelerated acid degradation
Scaffolds from the 3, 20 mm/s, and mineralization (20 mm/s) groups were immersed in 15 mL 5 M HCL and maintained at 37°C to mimic bacterial infection conditions in vivo. Thereafter, the scaffolds were carefully rinsed with deionized water to remove the residual HCL until the pH became neutral (i.e., pH 7), before freeze-drying for 36 h. The dry weights of the scaffolds were measured for comparison with the original state. The experiments were performed in triplicate for each group. The degradation rate was expressed as weight loss using Equation (IV):
where m0 and m represent the scaffold weights before and after acidic immersion, respectively.
2.9. Hydrophilic angle experiment
The scaffold surface was cleaned with anhydrous ethanol and a lint-free cloth to remove contaminants. The sample was fixed on a glass slide, and a droplet of water was deposited on the sample surface. The contact angle measuring instrument (JY-82C, ChengDeDengSheng, China) was used to analyze the droplet image using the instrument’s built-in software, capturing an image every 62 ms. The contact angle was measured from each image at the moment the droplet first contacted the sample. This measurement was repeated three times for each group of scaffolds, and the mean was calculated accordingly.
2.10. Cell viability
Human umbilical vein endothelial cells (HUVECs) were obtained from the China Center for Type Culture Collection (Wuhan University, China). HUVECs were cultured in DMEM/F-12 cell medium (Wuhan Servicebio Technology, China), supplemented with 10% fetal bovine serum (FBS; Procell, China). The cells were cultured in a cell incubator (37°C; 5% CO2). At approximately 90% confluency, the cells were passaged. HUVECs (5 × 104 cells/scaffolds) were inoculated in different sterilized scaffolds (12 × 12 × 3 mm3), placed in a 24-well plate for 1 h, and then cultured with 1 mL DMEM/F-12 medium. The growth was measured using calcein-AM and propidium iodide (PI) staining.
2.11. Statistical analysis
The data were expressed as the mean ± standard deviation and were analyzed using one-way analysis of variance (ANOVA). A p-value < 0.05 was considered statistically significant.
3. Results
3.1. Cryogenic 3D printing process of cross-scale collagen scaffold
The temperature distribution of the printing slurry during preparation was recorded using an infrared camera (Figure 3). It was found that the low-temperature field was affected by the printing velocity. Specifically, at the same size, when the printing speed was slow (3 mm/s), only one fiber was above 0°C, which could be associated with adequate fiber cooling. When the slurry was printed into a fiber, the previously printed fibers had already been cooled. At a speed of 3 mm/s, the low-temperature gradient displayed two patterns: (i) vertical direction (from bottom to top), likely due to the cold source of frozen fibers in the lower layer; and (ii) horizontal direction (from left to right), likely due to the cold source of frozen fiber in the same layer. At a high printing speed (20 mm/s), the fibers in an entire layer remained above 0°C. This could be associated with the short heat transfer time during the cooling stage. As a result, fibers in the same layer had not yet frozen. In this situation, the low-temperature gradient displayed only the vertical pattern (from bottom to top) due to the cold source of frozen fibers in the lower layer.
Figure 3. Infrared imaging of temperature distribution during scaffold printing at different speeds.
Figure 4 displays the distribution of isothermal surfaces and temperature (T) streamlines at different time points and speeds (3 and 20 mm/s). The light blue T streamlines are perpendicular to the isothermal surfaces, representing the low-temperature gradients. Furthermore, ice crystals were observed to grow along the low-temperature gradient. Fiber formation at a printing speed of 20 mm/s occurred much faster than at 3 mm/s. As a result, the temperature field in the 20 mm/s group stabilized at 0.2 s. At this point, the entire fiber had already been extruded at 20 mm/s, with T streamlines oriented vertically. In contrast, at 3 mm/s, fiber extrusion had not been completed, and the isothermal surfaces were much closer due to the longer cooling time, causing the T streamlines to be horizontal. In other words, the low-temperature field can be modulated by adjusting the printing speed, allowing for controlled microstructure formation. These simulation results provided insights into the direction and shape of ice crystals, which were further validated using SEM and computed tomography (CT) imaging.
Figure 4. Simulation of the isothermal surface and temperature streamlines at different time points and printing speeds (3 and 20 mm/s).
3.2. Microstructure of the cryogenic 3D-printed collagen scaffold
The cryogenic 3D-printed scaffold features a cross-scale structure with high porosity. The formation mechanism depends on the water in the slurry freezing into ice crystals due to the low-temperature field during the printing process. The ice crystals are then removed through lyophilization, forming micropores inside the fibers, thereby creating a cross-scale structure with high porosity. Likewise, the cryogenic-printed scaffold can be regarded as a type of aerogel, possessing high porosity and low density (Figure 5a). As observed, the lower fibers serve as cryogenic sources for the upper fibers, forming radial lamellas inside the scaffold fibers. To investigate the influence of the low-temperature field on the microstructure of the scaffolds, the scaffold cross-section was divided into following two regions: (i) a region not in contact with fibers from other layers, designated as the overhang region (Figure 5b and d); and (ii) an overlapped region in contact with fibers from other layers, designated as the contact region (Figure 5c and e). Due to the low-temperature gradient from the cold source (mainly the frozen fibers), the micropores are formed in lamella shape and the radiation direction, mimicking a native lamellar bone.
Figure 5. Scanning electron microscopy (SEM) images of the microstructure at different areas of the porous scaffold. (a) Cross-section image and overall structure (a, inset) of the cryogenic-printed scaffold. (b) Cross-section image and overall structure (b, inset) of the overhang region. (c) Cross-section image and overall structure (c, inset) of the contact region. (d) Surface and overall structure (d, inset) of the overhang region. (e) Surface and overall structure (e, inset) of the contact region. Scale bars: 100 µm.
3.3. Microstructure control of cryogenic 3D-printed collagen scaffolds
Figure 6a illustrates the microstructure of cryogenic 3D-printed scaffolds under different printing speeds. At a speed of 3 mm/s, due to the horizontal low-temperature gradient (Figure 3), the left part of the overhang region displays evident horizontal lamellas (H). In the right part of the overhang region, the lamella shape shifts to a vertical orientation as the extruded fibers attach to the new cold resource (V). Below the H lamellas, some amorphous areas were observed in the cross-section images. When the speed increased to 5 mm/s, the ice crystal growth time decreased, leading to a reduction in the amorphous area below the H lamellas. Furthermore, when the speed increased to 20 mm/s, the extruded fibers formed before freezing, resulting in a vertical low-temperature gradient from bottom to top. Consequently, the H area decreased, while the V area increased. This finding was verified through CT imaging (Figure 6b), which aligned with the simulation predictions.
Figure 6. The micro-structure of cryogenic 3D printed scaffold. (a) Scanning electron microscopy (SEM) images of the microstructure of porous scaffolds under different printing speeds. (b) Computed tomography (CT) images of the microstructure of porous scaffolds under 3 mm/s. The red boxes and marks show the H and V part form the front view and top view. Abbreviations: H, horizontal lamellas; V, vertical lamellas. Scale bars: 100 µm (a).
3.4. In situ mineralization of cryogenic 3D printed collagen scaffolds
Figure 7 demonstrates that in situ mineralization did not influence the microstructure of the cryogenic-printed scaffold. It was observed that calcium mineral was embedded within the micro-lamellas. Additionally, the SEM images indicate the presence of calcium mineral crystals on the surface of the scaffold in the overhang region (indicated by arrows in Figure 7b). Additionally, some of the pores on the surface of the scaffold were covered with crystals. The mineralization process did not damage the microstructure of the scaffolds, which could be attributed to PAA maintaining the calcium ions in the precursor state and facilitating their entry into the collagen fibers.20 The energy dispersive spectrometry (EDS) results demonstrate the presence of calcium on the scaffold surface and lamella walls. The distribution of Ga element largely overlapped with carbon, which further substantiates the efficacy of the in situ mineralization process. In this study, different printing speeds were used to obtain scaffolds with different microstructures at the overhang region, resulting in different mineralization effects. Furthermore, it was reported that the mineralized twisted plywood pattern could stimulate bone formation and enhance performance.29
Figure 7. Scanning electron microscopy (SEM) images and energy dispersive spectrometry (EDS) spectra of the scaffolds (a) before and (b) after mineralization. Scale bars: 100 µm (leftmost; left); 10 µm (right).
3.5. Chemical structure of collagen scaffolds before and after mineralization
In an FTIR spectrum, the absorption peaks of carbonate compounds are typically observed within the range of 1200–1800 cm−1, which corresponds to the vibrations of the C–O and C=O bonds in carbonates. In particular, peaks situated at approximately 1200 cm−1 indicate the vibration of the C–O bond, whereas the peaks located at 1600–1800 cm−1 represent the vibration of the C=O bond. From the FTIR spectra (Figure 8a), mineralized scaffolds exhibited more substantial absorption peaks for the C–O and C=O bonds than the untreated scaffolds, thereby substantiating the generation of carbonates within the scaffolds. The thermogravimetric results (Figure 8b) suggest the presence of calcium carbonate in the scaffolds and indicate that the printing speed did not affect the mineralization outcomes. The scaffolds are composed of calcium carbonate (melting point: 70–100°C), calcium chloride (772°C), sodium chloride (801°C), sodium carbonate (851°C), and calcium oxide (1339°C), along with other compounds with melting points below 1000°C. As the temperature increases to 1000°C, materials with melting points below 1000°C progressively melt and disappear. Consequently, the remaining material can be identified as calcium carbonate.
Figure 8. Composition of the scaffold. (a) Fourier transform infrared (FTIR) spectra of the scaffolds. (b) Thermogravimetric analysis of the scaffolds. Scaffold A represents the untreated scaffolds; scaffold B represents the mineralized scaffolds.
3.6. Mechanical properties of mineralized cryogenic 3D-printed collagen scaffolds
The tensile modulus of collagen scaffolds printed at a speed of 20 and 3 mm/s were measured as 3.358 ± 0.85 and 0.684 ± 0.092 MPa, respectively (Figure 9a). Tensile modulus was increased by 392.6% with the increase in printing speed from 3 to 20 mm/s. As illustrated in Figure 9a, the tensile curve at 20 mm/s approaches zero growth of tensile stress when the tensile deformation is 10%. During scaffold stretching, the distance between the upper and lower tensile sheets becomes smaller than the scaffold height. Consequently, a slight compression occurs prior to scaffold stretching, resulting in zero tensile stress growth after the scaffold reaches its designated height. The Young’s modulus of collagen scaffolds printed at a speed of 20 and 3 mm/s were 0.595 ± 0.02 and 0.404 ± 0.01 MPa, respectively (Figure 9b). Young’s modulus was increased by 147.5% with the increase in printing speed from 3 to 20 mm/s. As illustrated in Figure 9d, the vertical collagen lamellas could withstand compression. When the scaffold was subjected to pressure, the lamellar walls gradually bent at 50% compression before collapsing at 90% compression. Furthermore, when the compression was lower than 60%, the structure of the micro-lamellas was able to recover. Mineralization was adopted in the post-treatment to enhance the mechanical properties of the collagen scaffold. The Young’s modulus of the two groups of scaffolds exhibited a notable increase (Figure 9b). The Young’s modulus of the scaffold with a printing speed of 20 mm/s (1.36 ± 0.1 MPa) was increased by twofolds compared to that of the scaffold with a printing speed of 3 mm/s (0.595 ± 0.02 MPa). After microstructure control and in situ mineralization, Young’s modulus of the cryogenic-printed scaffold increased by 240%. It was demonstrated in Figure 9c that mineralization did not damage the high porosity of the cryogenic-printed collagen scaffold. In comparison with previous studies, this cryogenic-printed collagen/ Ca scaffold exhibited excellent compressive modulus and porosity, reaching a compressive modulus of 1.36 MPa and porosity of 95% (Table S1, Supporting Information); the Young’s modulus of the previously reported collagen/Ca scaffolds was typically lower than 500 kPa.
Figure 9. The mechanical test and micro-structure change of cryogenic 3D printed scaffold. (a) Tensile curves (upper), and tensile modulus (lower) of scaffolds under different printing speeds, inset: the tensile test of scaffold. (b) Compression curves (upper), compressive modulus (lower) of scaffolds under different printing speeds, before and after mineralization (M), inset: the compression test of scaffold. 3+0, 20+0: Scaffold printed under speeds of 3 and 12 mm/s before mineralization. 3+10, 20+10: Scaffold printed under speeds of 3 and 12 mm/s after mineralization for 10 h. (c) The porosity of cryogenic-printed scaffold before and after mineralization (upper). The comparison of Young’s modulus and porosity between the presented scaffold in this papers and previous scaffolds. (d) Cross-section images of micro-structures (upper) and whole scaffold (lower) at different compression degrees. **p < 0.005; ***p < 0.001; scale bars: 100 µm (d).
3.7. Falling-ball impact experiment
When the ball was dropped from a height of 0.3 m, the rebound height of the unmineralized scaffold was 0.035 m, and the energy loss was 0.1325 J; while the rebound height of the mineralized scaffold was 0.01 m, and the energy loss was 0.145 J. When the ball was dropped from a height of 0.5 m, the rebound height of the unmineralized scaffold was 0.015 m, and the energy loss was 0.2425 J; while the mineralized scaffold was shattered, and the energy loss was 0.25 J. When the ball was dropped from a height of 0.7 m, the unmineralized scaffold barely rebounded, and the energy loss was 0.35 J; since the mineralized scaffold had already been shattered at the drop height of 0.5 m, no further experiments were conducted at the height of 0.7 m. In summary, the energy absorption limit of the unmineralized scaffold is 0.35 J; after mineralization, the scaffold’s energy absorption limit is 0.25 J (Figure S3, Supporting Information).
The falling-ball impact tests were utilized to examine the mechanical properties of both unmineralized and mineralized samples under dynamic impact conditions (Figure 10). When a steel ball was dropped from a height of 0.5 m onto the samples, the unmineralized samples remained intact; however, the mineralized samples were broken into numerous pieces. When the ball fell onto an unmineralized sample, the energy from the fall caused the ball to bounce back into the air. In contrast, when the ball fell onto the mineralized sample, the ball impacted the sample, breaking it into numerous pieces without bouncing (Figure 10e and f; Videos S1 and S2, Supporting Information). The surface flatness of the unmineralized scaffolds was further evaluated using a super depth of field camera (DSX1000, Olympus, China). The results indicate that the falling ball created a crater on the scaffold of approximately 1245.7 μm in diameter, suggesting that the scaffold had some impact resistance (Figures 10b and S2, Supporting Information). These results demonstrate that mineralization alters the energy absorption mechanism of the samples. The unmineralized samples primarily exhibit elastic and plastic deformation after impact, returning a portion of the energy to the impacting ball. Conversely, the energy absorption mechanism of the mineralized samples is predominantly characterized by the generation and propagation of cracks within the material. This indicates that mineralization can transform the mechanical behavior of the samples from plastic to rigid.
Figure 10. Falling-ball impact tests. (a and b) Images of the unmineralized samples before (a) and after (b) the ball impact. (c and d) Images of the mineralized samples before (c) and after (d) the ball impact. (e and f) Ball rebound upon impact on unmineralized (e) and mineralized (f) samples.
3.8. Accelerated acidic degradation
The degradation results are presented in Figure 11a. During the experiment, all scaffolds did not completely degrade. After 2 h of immersion, the degradation rates of the scaffolds with printing speeds of 3 and 20 mm/s were 20.75 ± 7.63% and 23.84 ± 10.67%, respectively. Meanwhile, that of the mineralized scaffolds was 21.99 ± 1.21%. At this stage, neither the printing speed nor the mineralization process had a significant impact on the scaffold degradation rate, all of which were approximately 11%/h. In the first 2 h, the scaffolds degraded at a rate of roughly 11%/h, and this was when the degradation rate of the scaffolds was the fastest.
Figure 11. The degradation of cyrogenic printed scaffolds. (a) Weight loss curve of the printed scaffold in the accelerated acidic degradation experiment. (b) Comparison of the scaffolds before and after 10 h of immersion: I represents the scaffold with a print speed of 3 mm/s; II represents the scaffold with a print speed of 20 mm/s; and III represents the scaffold after mineralization with a print speed of 20 mm/s.
After 6 h, the degradation rates of the scaffolds with printing speeds of 3 and 20 mm/s reached 36.81 ± 6.01% and 35.66 ± 2.13%, respectively, while the degradation rate of the mineralized scaffolds was 50.00 ± 7.14%. Within 2–6 h, the degradation rates of the scaffolds began exhibiting notable differences, with the mineralized scaffolds degrading the fastest at a rate of approximately 6.5%/h; likely associated with HCl penetrating the scaffold, corroding part of the collagen by reacting with the inorganic calcium salts within the collagen fibers. Consequently, this resulted in a more substantial reduction in the scaffold mass. During this time, the effect of printing speed on the scaffold degradation was minimal, with the degradation rate of the two groups of scaffolds at approximately 4%/h. The printing speed has little effect on the basic degradation rate because the porosity of the scaffolds prepared with the two parameters did not vary significantly, and the raw materials of the two scaffolds were identical.
After 10 h, the degradation rates of the scaffolds with printing speeds of 3 and 20 mm/s were 40.21 ± 0.36% and 41.89 ± 2.01%, respectively; while the degradation rate of the mineralized scaffolds was 56.04 ± 1.90%. Within 6–10 h, the degradation rates of the three groups of scaffolds displayed minimal differences (i.e., approximately 1%/h), as the material and porosity of the three groups of scaffolds remained largely similar after 6 h of corrosion.
Notably, after 10 h of corrosion, the unmineralized scaffolds shrank and deformed, while the mineralized scaffolds did not exhibit any deformation (Figure 10b). Hence, it can be inferred that the mineralized inorganic calcium salts protected the collagen to a certain extent, making it more corrosion-resistant.30
3.9. Hydrophilic angle experiment
The hydrophilic angle experiments revealed that the contact angle of the unmineralized scaffold was 46.9°, while the contact angle of the mineralized scaffold was 35.91° (Figure 12). The contact angle of the unmineralized scaffold is consistent with the findings of previous studies. The decrease in the water contact angle of the mineralized scaffold indicates an increase in its hydrophilicity. This phenomenon occurs because, during the mineralization process, inorganic minerals (carbonates) are deposited on the surface of the collagen, introducing more hydrophilic groups (such as hydroxyl and carbonate groups).31 These groups enhance the surface hydrophilicity, thereby reducing the water contact angle. Additionally, after mineralization, the surface energy of the collagen increases, particularly the polar component. Materials with higher surface energy interact more readily with water molecules, leading to a decrease in the water contact angle.
Figure 12. Hydrophilic angle of (a) unmineralized scaffolds and (b) mineralized scaffolds.
3.10. Cell viability
As displayed in Figure 13, HUVECs were successfully cultured on the unmineralized and mineralized collagen scaffolds, indicating the biocompatibility and non-toxicity of the scaffolds. The calcein-AM assay was conducted on the HUVECs seeded on scaffolds. Live cells (green) appeared to adhere to the scaffolds. No dead cells were observed. Collagen, calcium minerals, and collagen with intrafibrillar mineralization are all natural biomaterials, known for their excellent biocompatibility. Our findings are consistent with previous studies.32
Figure 13. Calcein-AM staining of human umbilical vein endothelial cells (HUVECs) cultured for 1 and 3 days on (a) unmineralized scaffolds and (b) mineralized scaffolds. Scale bars: 100 µm.
4. Discussion
Large bone defect repair remains a challenge in the clinical setting. Autograft transplantation is the gold standard treatment strategy, but it often faces challenges such as donor shortages and size limitations. Therefore, there is a significant demand for artificial cancellous bone. TE scaffolds are a promising alternative and have attracted increasing attention recently.1,2 The ideal TE scaffold should possess natural bone biomimetic material components, structure, and mechanical properties, providing a similar environment and stimulation cues for cell growth and bone tissue regeneration.3,4 The structure of the bone TE scaffold plays a vital role in tissue regeneration to provide mechanical and topological cues (durotaxis and topotaxis) for cell growth and differentiation.8,33 Natural bone has a cross-scale structure (ranging from cm to μm) that confers high porosity and specific surface area while maintaining adequate mechanical strength. Mechanistically, collagen and calcium minerals in bone assemble at the micron level, significantly enhancing stiffness at a high porosity (>90%). However, due to the fabrication precision limit, existing mineralized collagen bone scaffolds can hardly realize the cross-scale structure. Thus, the porosity and strength of these scaffolds cannot match those of natural bone. For example, a 3D-printed scaffold with a dimensional accuracy of 0.1 mm can prevent mineral ions from entering the scaffold fiber, consequently resulting in poor mechanical properties (e.g., Young’s modulus within the kPa range).34 In contrast, casting scaffolds through molds is a direct method, and in some cases, a low-temperature field is integrated into the casting process (freeze-casting), such as in the fabrication of porous collagen/HA composite scaffolds.23 The advantage of freeze casting is the feasible adjustment of the collagen–HA ratio, but there is limited control of the scaffold shape.
Cryogenic 3D printing is a low-temperature field-assisted fabrication process. During fiber formation, the ice wall serves as a template, forcing the collagen into the form of lamellas with a large specific surface area and interconnecting pores. These features make cryogenic-printed porous scaffolds a promising candidate for bone TE scaffolds. The interconnecting pores and high porosity can facilitate blood perfusion and nutrient exchange.35 In addition, the large specific surface area is beneficial for drug loading. Furthermore, the fast freezing process can form an overhang structure without support, and the low temperature is suitable for printing live cell-laden bioink.36 However, cryogenic porous scaffolds are regarded as poor drug-loading platforms due to their poor mechanical strength caused by high porosity.37
To address this issue, this study controlled the microstructure of scaffolds to enhance the mechanical properties. Firstly, the shape of collagen lamella was determined by the shape of the ice crystals, which was regulated by the temperature gradient. During cryogenic printing, the frozen fibers function as cold sources for the printing slurry. Additionally, nozzle movement speed affects the collagen lamella direction, which subsequently regulates the stiffness. As a result, Young’s modulus of cryogenic 3D-printed scaffold increased by 1.5 times through adjustments of the collagen micro-lamellar angle.
Secondly, the high surface area of the lamellar scaffold enables in situ mineralization. In natural bone, calcium phosphate crystals form inside the collagen fibrils. Researchers found that the amorphous liquid-phase mineral precursor phase could enter into the nano-gap and grooves of collagen fibrils and then crystallize into stable minerals, embedding inside collagen fibrils.17 With the incorporation of PAA, the mineral ions can remain amorphous and mineralize in the fibrils.38 In this study, in situ mineralization was applied as post-treatment, increasing Young’s modulus of the cryogenic 3D-printed scaffold by twofolds. The combination of microstructure adjustment and in situ mineralization enhanced the Young’s modulus of a highly porous scaffold (porosity: 95%) by threefolds, reaching 1.36 MPa, which aligns with that of cancellous bone.
5. Conclusion
Inspired by the cross-scale structure of natural bone, this study adopted cryogenic 3D printing to fabricate a cross-scale, mineralized collagen bone TE scaffold with high porosity. The microstructure of the scaffold was regulated by adjusting the low-temperature gradient. Furthermore, the in situ mineralization was adopted as post-treatment. By combining micro-lamellar pattern control and in situ mineralization, Young’s modulus of cryogenic 3D-printed scaffold increased by 240%. The obtained cross-scale scaffold possessed a porosity of 95% and Young’s modulus of 1.36 MPa, similar to the properties of natural bone, making it a promising bone repair candidate. Future research should focus on two key areas: (i) a deeper investigation into the mechanism of ice crystal growth, as current understanding remains limited; and (ii) overcoming the challenges of fabricating more complex structures using cryogenic 3D printing, despite its success in producing lamellar microstructures. In addition, due to the high porosity of scaffolds fabricated with this technology, they hold potential for applications in impact resistance, wave absorption, thermal insulation, flexible materials, piezoelectric ceramics, and more.
1.Dixit K, Vishwakarma A, Kumar H, Kim K, Sinha N. Material extrusion additive manufacturing of graphene oxide reinforced 13–93B1 bioactive glass scaffolds for bone tissue engineering applications. Addit Manuf. 2024;94:104481.
doi: 10.1016/j.addma.2024.104481
2.Wang Y, Pereira RF, Peach C, Huang B, Vyas C, Bartolo P. Robotic in situ bioprinting for cartilage tissue engineering. Int J Extrem Manuf. 2023;5(3):032004.
3.Chen A, Su J, Li Y, et al. 3D/4D printed bio-piezoelectric smart scaffolds for next-generation bone tissue engineering. Int J Extrem Manuf. 2023;5(3):032007.
4.Zhou X, Zou B, Chen Q, et al. Polyelectrolyte multilayer coating on 3D printed PEGDA/TCP scaffold with improved cell proliferation. Addit Manuf Front. 2024;3(1):200114.
5.Reznikov N, Shahar R, Weiner S. Bone hierarchical structure in three dimensions. Acta Biomater. 2014;10(9):3815-3826.
doi: 10.1016/j.actbio.2014.05.024
6.Reznikov N, Bilton M, Lari L, Stevens MM, Kroeger R. Fractal-like hierarchical organization of bone begins at the nanoscale. Science. 2018;360(6388):eaao2189.
7.Mao A, Zhao N, Liang Y, Bai H. Mechanically efficient cellular materials inspired by cuttlebone. Adv Mater. 2021;33(15):e2007348.
8.Sengupta S, Parent CA, Bear JE. The principles of directed cell migration. Nat Rev Mol Cell Biol. 2021;22(8): 529-547.
doi: 10.1038/s41580-021-00366-6
9.Zhang Y, Xing Y, Li J, et al. Osteogenesis-related behavior of MC3T3-E1 cells on substrates with tunable stiffness. Biomed Res Int. 2018;2018:4025083.
doi: 10.1155/2018/4025083
10.Xie J, Zhang D, Zhou C, Yuan Q, Ye L, Zhou X. Substrate elasticity regulates adipose-derived stromal cell differentiation towards osteogenesis and adipogenesis through β-catenin transduction. Acta Biomater. 2018;79:83-95.
doi: 10.1016/j.actbio.2018.08.018
11.Guo Y, Qiao Y, Quan S, Yang C, Li J. Relationship of matrix stiffness and cell morphology in regulation of osteogenesis and adipogenesis of BMSCs. Mol Biol Rep. 2022;49(4):2677-2685.
doi: 10.1007/s11033-021-07075-5
12.Chen J-y, Wang Y-x, Ren K-f, Wang Y-b, Fu G-s, Ji J. The influence of substrate stiffness on osteogenesis of vascular smooth muscle cells. Colloids Surf B: Biointerfaces. 2021;197:111388.
doi: 10.1016/j.colsurfb.2020.111388
13.Vallet-Regí M, González-Calbet JM. Calcium phosphates as substitution of bone tissues. Prog Solid State Chem. 2004;32(1-2):1-31.
doi: 10.1016/j.progsolidstchem.2004.07.001
14.Yang C, Li GY. A biomimetic mineralized collagen hydrogel containing uniformly distributed and highly abundant dopamine-modified hydroxyapatite particles for bone tissue engineering. J Appl Polymer Sci. 2024;141(26):e55567.
doi: 10.1002/app.55567
15.Silva CC, Thomazini D, Pinheiro AG, et al. Collagenhydroxyapatite films: piezoelectric properties. Mater Sci Eng B. 2001;86(3): 210-218.
doi: 10.1016/s0921-5107(01)00674-2
16.Landis WJ, Jacquet R. Association of calcium and phosphate ions with collagen in the mineralization of vertebrate tissues. Calcif Tissue Int. 2013;93(4):329-337.
doi: 10.1007/s00223-013-9725-7
17.Olszta MJ, Cheng X, Jee SS, et al. Bone structure and formation: a new perspective. Mater Sci Eng R. 2007;58(3):77-116.
doi: 10.1016/j.mser.2007.05.001
18.Wang Q-q, Miao L, Zhang H, Wang SQ, Li Q, Sun W. A novel amphiphilic oligopeptide induced the intrafibrillar mineralisation via interacting with collagen and minerals. J Mater Chem B. 2020;8(11):2350-2362.
doi: 10.1039/C9TB02928A
19.Gilbert PUPA, Bergmann KD, Boekelheide N, et al. Biomineralization: integrating mechanism and evolutionary history. Sci Adv. 2022;8(10):eabl9653.
20.Shen L, Bu H, Zhang Y, Tang P, Li G. Molecular weight and concentration of poly (acrylic acid) dual-responsive homogeneous and intrafibrillar collagen mineralization using an in situ co-organization strategy. Polymer Compos. 2021;42(9):4448-4460.
doi: 10.1002/pc.26161
21.Orgel JPRO, Miller A, Irving TC, et al. The in situ supermolecular structure of type i collagen. Structure. 2001;9(11):1061-1069.
doi: 10.1016/S0969-2126(01)00669-4
22.Landis WJ, Silver FH. The structure and function of normally mineralizing avian tendons. Compar Biochem Physiol A. 2002;133(4):1135-1157.
doi: 10.1016/S1095-6433(02)00248-9
23.Minardi S, Taraballi F, Cabrera FJ, et al. Biomimetic hydroxyapatite/collagen composite drives bone niche recapitulation in a rabbit orthotopic model. Mater Today Bio. 2019;2:100005.
doi: 10.1016/j.mtbio.2019.100005
24.Guo C, Wu J, Zeng Y, Li H. Construction of 3D bioprinting of HAP/collagen scaffold in gelation bath for bone tissue engineering. Regen Biomater. 2023;10:rbad067.
doi: 10.1093/rb/rbad067
25.Jiao Z, Luo B, Xiang S, Ma H, Yu Y, Yang W. 3D printing of HA/PCL composite tissue engineering scaffolds. Adv Ind Eng Polym Res. 2019;2(4):196-202.
doi: 10.1016/j.aiepr.2019.09.003
26.Hu Y, Wu B, Xiong Y, et al. Cryogenic 3D printed hydrogel scaffolds loading exosomes accelerate diabetic wound healing. Chem Eng J. 2021;426:130634.
doi: 10.1016/j.cej.2021.130634
27.Sun T, Meng C, Ding Q, et al. In situ bone regeneration with sequential delivery of aptamer and BMP2 from an ECM-based scaffold fabricated by cryogenic free-form extrusion. Bioact Mater. 2021;6(11):4163-4175.
doi: 10.1016/j.bioactmat.2021.04.013
28.Ye J, Zhou X, Huang Z, et al. Low-temperature-field-assisted fabrication of cross-scale tissue engineering scaffolds. Int J Extrem Manuf. 2025;7(2):022011.
29.Robin M, Mouloungui E, Castillo G, et al. Mineralized collagen plywood contributes to bone autograft performance. Nature. 2024;636(8041):100-107.
doi: 10.1038/s41586-024-08208-z
30.Liu T, Yang B, Tian W, Zhang X, Wu B. Cryogenic coaxial printing for 3D shell/core tissue engineering scaffold with polymeric shell and drug-loaded core. Polymers (Basel). 2022;14(9):1722.
31.Juárez-Moreno JA, Ávila-Ortega A, Oliva AI, Avilés F, Cauich-Rodríguez JV. Effect of wettability and surface roughness on the adhesion properties of collagen on PDMS films treated by capacitively coupled oxygen plasma. Appl Surf Sci. 2015;349:763-773.
doi: 10.1016/j.apsusc.2015.05.063
32.Thrivikraman G, Athirasala A, Gordon R, et al. Rapid fabrication of vascularized and innervated cell-laden bone models with biomimetic intrafibrillar collagen mineralization. Nat Commun. 2019;10(1):3520.
doi: 10.1038/s41467-019-11455-8
33.Deng B, Huang Z, Zhang X, et al. Durotaxis and topotaxis orchestrated guidance on cell migration in 3D printed scaffold/hydrogel composite. Add Manuf Front. 2024;3:200134.
doi: 10.1016/j.amf.2024.200134
34.Lee H, Yang GH, Kim M, Lee J, Huh J, Kim G. Fabrication of micro/nanoporous collagen/dECM/silk-fibroin biocomposite scaffolds using a low temperature 3D printing process for bone tissue regeneration. Mater Sci Eng C. 2018;84:140-147.
doi: 10.1016/j.msec.2017.11.013
35.Shi L, Hu Y, Ullah MW, et al. Cryogenic free-form extrusion bioprinting of decellularized small intestinal submucosa for potential applications in skin tissue engineering. Biofabrication. 2019;11(3):035023.
36.Ravanbakhsh H, Luo Z, Zhang X, et al. Freeform cellladen cryobioprinting for shelf-ready tissue fabrication and storage. Matter. 2022;5(2):573-593.
doi: 10.1016/j.matt.2021.11.020
37.Lai J, Wang C, Liu J, et al. Low temperature hybrid 3D printing of hierarchically porous bone tissue engineering scaffolds with in situ delivery of osteogenic peptide and mesenchymal stem cells. Biofabrication. 2022;14(4):045006.
38.Ping H, Wagermaier W, Horbelt N, et al. Mineralization generates megapascal contractile stresses in collagen fibrils. Science. 2022;376(6589):188-192.