Effect of PCL/HA material characteristics on the degradation and mechanical properties of interbody fusion cages
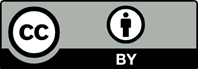
Interbody fusion cages are key implants in spinal interbody fusion surgeries. For the novel biodegradable interbody fusion cages that can be gradually absorbed and replaced in the body, the formulation system of the materials plays a crucial role in their long-term biocompatibility and mechanical stability. In this study, PCL/ HA composites with high hydroxyapatite (HA) mass fractions (25, 30, 35, 40%) and different polycaprolactone (PCL) molecular weights (50 and 80 kDa) were fabricated. Biodegradable PCL/HA interbody fusion cages of different material systems were printed by polymer melt differential three-dimensional (3D) printing technology. In vitro degradation tests were conducted in a simulated in vivo environment to quantitatively analyze the stability and degradation behavior of PCL/HA interbody fusion cages with different material compositions and molecular weights in an artificially simulated body fluid environment, and their mechanical properties under loads. The cytotoxicity assays of the material were also conducted. The findings revealed that the degradation rate of the interbody fusion cage accelerated as the HA content increased. The strength and stiffness of each material system exceeded the minimum requirement for human cancellous bone. When the HA content was the same, the degradation rate of the high molecular weight (80 kDa) interbody fusion cage was faster compared to that of the 50 kDa molecular weight cage. When the HA mass fraction was below 30wt%, the compressive strength and compressive modulus of the fusion cage increased with increasing HA content. However, when the HA mass fraction exceeded 30wt%, the mechanical properties of the fusion cage worsened with higher HA content. A comparative analysis of material properties indicated that when the HA content was 30wt%, the fusion cage exhibited a moderate degradation rate, high compressive strength and modulus, and excellent 3D printing performance, making it the formulation system with the best overall performance. In addition, the PCL material, which was also used in most literature, exhibited varying levels of cytotoxicity depending on the cytotoxicity testing method. Further animal implantation experiments of spinal fusion cages require the selection of medical-grade implant materials.

- Wei X, Han T, Qi B, et al. Thinking and practice of integrated Chinese and Western medicine for the treatment of degenerative diseases of the spine. China J Orthop Traumatol. 2023;36(04):345-347. doi: 10.12200/j.issn.1003-0034.2023.04.009
- Verma R, Virk S, Qureshi S. Interbody fusions in the lumbar spine: a review. HSS J. 2020;16(2):162-167. doi: 10.1007/s11420-019-09737-4
- Mobbs RJ, Phan K, Malham G, Seex K, Rao PJ. Lumbar interbody fusion: techniques, indications and comparison of interbody fusion options including PLIF, TLIF, MI-TLIF, OLIF/ATP, LLIF and ALIF. J Spine Surg. 2015;1(1):2-18. doi: 10.3978/j.issn.2414-469X.2015.10.05
- Laubach M, Kobbe P, Hutmacher DW. Biodegradable interbody cages for lumbar spine fusion: current concepts and future directions. Biomaterials. 2022;288:121699. doi: 10.1016/j.biomaterials.2022.121699
- Koutserimpas C, Alpantaki K, Chatzinikolaidou M, Chlouverakis G, Dohm M, Hadjipavlou AG. The effectiveness of biodegradable instrumentation in the treatment of spinal fractures. Injury. 2018;49(12):2111-2120. doi: 10.1016/j.injury.2018.11.008
- Daskalakis E, Liu F, Huang B, et al. Investigating the influence of architecture and material composition of 3D printed anatomical design scaffolds for large bone defects. Int J Bioprint. 2021;7(2):268. doi: 10.18063/ij;b.v7i2.268
- Han X, Gao Y, Ding Y, et al. In vitro performance of 3D printed PCL −β -TCP degradable spinal fusion cage. J Biomater Appl. 2021;35(10):1304-1314. doi: 10.1177/0885328220978492
- Lewandrowski KU, Vira S, Elfar JC, Lorio MP. Advancements in custom 3D-printed titanium interbody spinal fusion cages and their relevance in personalized spine care. J Pers Med. 2024;14(8):809. doi: 10.3390/jpm14080809
- Donaldson C, Santro T, Awad M, Morokoff A. 3D-printed titanium alloy cage in anterior and lateral lumbar interbody fusion for degenerative lumbar spine disease. J Spine Surg. 2024;10(1):22-29. doi: 10.21037/jss-23-120
- Takeuchi S, Inoue T, Takahashi T, Kanematsu R, Minami M, Hanakita J. The effectiveness of tritanium cages in preventing osteolytic vertebral endplate cysts after lumbar interbody fusion. World Neurosurg. 2024;184: e803-e808. doi: 10.1016/j.wneu.2024.02.055
- Kersten RF, van Gaalen SM, de Gast A, Öner FC. Polyetheretherketone (PEEK) cages in cervical applications: a systematic review. Spine J. 2015;15(6):1446-1460. doi: 10.1016/j.spinee.2013.08.030
- Tan JH, Cheong CK, Hey HWD. Titanium (Ti) cages may be superior to polyetheretherketone (PEEK) cages in lumbar interbody fusion: a systematic review and meta-analysis of clinical and radiological outcomes of spinal interbody fusions using Ti versus PEEK cages. Eur Spine J. 2021;30(5):1285-1295. doi: 10.1007/s00586-021-06748-w
- Adl Amini D, Okano I, Oezel L, et al. Evaluation of cage subsidence in standalone lateral lumbar interbody fusion: novel 3D-printed titanium versus polyetheretherketone (PEEK) cage. Eur Spine J. 2021;30(8):2377-2384. doi: 10.1007/s00586-021-06912-2
- Chou YC, Chen DC, Hsieh WA, et al. Efficacy of anterior cervical fusion: comparison of titanium cages, polyetheretherketone (PEEK) cages and autogenous bone grafts. J Clin Neurosci. 2008;15(11): 1240-1245. doi: 10.1016/j.jocn.2007.05.016
- Phan K, Pelletier MH, Rao PJ, Choy WJ, Walsh WR, Mobbs RJ. Integral fixation titanium/polyetheretherketone cages for cervical arthrodesis: evolution of cage design and early radiological outcomes and fusion rates. Orthop Surg. 2019;11(1):52-59. doi: 10.1111/os.12413
- Karikari IO, Jain D, Owens TR, et al. Impact of subsidence on clinical outcomes and radiographic fusion rates in anterior cervical discectomy and fusion: a systematic review. J Spinal Disord Tech. 2014;27(1):1-10. doi: 10.1097/BSD.0b013e31825bd26d
- Seaman S, Kerezoudis P, Bydon M, Torner JC, Hitchon PW. Titanium vs. polyetheretherketone (PEEK) interbody fusion: Meta-analysis and review of the literature. J Clin Neurosci. 2017;44:23-29. doi: 10.1016/j.jocn.2017.06.062
- Williams D F. Assessing the triad of biocompatibility, medical device functionality and biological safety. Med Devices Sens. 2021;4(1):e10150. doi: 10.1002/mds3.10150
- Zhu L, Liu J, Bao X, Xu G, Liu Z, Zhao Y. Research advances in biodegradable intervertebral fusion cage for spine. J Reg Anat Oper Surg. 2019;28(1):71-75. doi: 10.11659/jjssx.08E018050
- Senaysoy S, Ilhan R, Lekesiz H. Mechanical deviation in 3D-printed PLA bone scaffolds during biodegradation. Comput Biol Med. 2024;183:109227. doi: 10.1016/j.compbiomed.2024.109227
- Kundak H, Bilisik K. Development of three-dimensional (3D) biodegradable polyglycolic acid fiber (PGA) preforms for scaffold applications: experimental patterning and fiber volume fraction-porosity modeling study. Polymers (Basel). 2023;15(9):2083. doi: 10.3390/polym15092083
- Mirzavandi Z, Poursamar SA, Amiri F, Bigham A, Rafienia M. 3D printed polycaprolactone/gelatin/ordered mesoporous calcium magnesium silicate nanocomposite scaffold for bone tissue regeneration. J Mater Sci Mater Med. 2024;35(1):58. doi: 10.1007/s10856-024-06828-5
- Yu X, Li X, Xue D, et al. In vitro degradation and biomechanical study of 3D printed PCL/β-TCP degradable intervertebral fusion cage. Progress in Modern Biomedicine. 2021;21(06):1001-1007. doi: 10.13241/j.cnki.pmb.2021.06.001
- Cao L, Chen Q, Jiang L, et al. Bioabsorbable self-retaining PLA/nano-sized β-TCP cervical spine interbody fusion cage in goat models: an in vivo study. Int J Nanomedicine. 2017;12:7197-7205. doi: 10.2147/IJN.S132041
- Pant S, Vijayaraghavan R, Loganathan S, Valapa RB. 3D-bioprinted poly (lactic acid)/β-TCP/mesoporous silica scaffolds: an investigation on in-vitro bioactivity and osteogenesis characteristics. Mater Today Chem. 2024;40:102246. doi: 10.1016/j.mtchem.2024.102246
- Rezania N, Asadi-Eydivand M, Abolfathi N, Bonakdar S, Mehrjoo M, Solati-Hashjin M. Three-dimensional printing of polycaprolactone/hydroxyapatite bone tissue engineering scaffolds mechanical properties and biological behavior. J Mater Sci Mater Med. 2022;33(3):31. doi: 10.1007/s10856-022-06653-8
- Backes EH, Beatrice CAG, Shimomura KMB, et al. Development of poly (ε-polycaprolactone)/hydroxyapatite composites for bone tissue regeneration. J Mater Res. 2021;36:3050-3062. doi: 10.1557/s43578-021-00316-0
- Liu F, Kang H, Liu Z, et al. 3D printed multi-functional scaffolds based on poly(ε-caprolactone) and hydroxyapatite composites. Nanomaterials (Basel). 2021;11(9):2456. doi: 10.3390/nano11092456
- Wang F, Tankus EB, Santarella F, et al. Fabrication and characterization of PCL/HA filament as a 3D printing material using thermal extrusion technology for bone tissue engineering. Polymers (Basel). 2022;14(4):669. doi: 10.3390/polym14040669
- Zhou Y, Hutmacher DW, Varawan SL, Lim TM. In vitro bone engineering based on polycaprolactone and polycaprolactone–tricalcium phosphate composites. Polym Int. 2007;56(3):333-342. doi: 10.1002/pi.2138
- Huang L. Comparative Study of HA, BCP and TCP Three- Dimensional Scaffolds on Osteogenesis. Guangxi Medical University, Nanning, CN. 2017. doi: 10.7666/d.D00999914
- Li X, Yuan Y, Liu L, et al. 3D printing of hydroxyapatite/ tricalcium phosphate scaffold with hierarchical porous structure for bone regeneration. Bio-Des Manuf. 2020;3:15-29. doi: 10.1007/s42242-019-00056-5
- Venugopal J, Rajeswari R, Shayanti M. et al. Electrosprayed hydroxyapatite on polymer nanofibers to differentiate mesenchymal stem cells to osteogenesis. Biomater Sci. Polymer Edition 2013;24(2):170-184. doi: 10.1163/156856212X629845
- Choi WY, Kim HE, Koh YH. Production, mechanical properties and in vitro biocompatibility of highly aligned porous poly (ε-caprolactone)(PCL)/hydroxyapatite (HA) scaffolds. J Porous Mater. 2013;20:701-708. doi: 10.1007/s10934-012-9644-4
- Xu N, Ye X, Wei D, et al. 3D artificial bones for bone repair prepared by computed tomography-guided fused deposition modeling for bone repair. ACS Appl Mater Inter. 2014;6(17):14952-14963. doi: 10.1021/am502716t
- Shor L, Güçeri S, Wen X, Gandhi M, Sun W. Fabrication of three-dimensional polycaprolactone/hydroxyapatite tissue scaffolds and osteoblast-scaffold interactions in vitro. Biomaterials. 2007;28(35):5291-5297. doi: 10.1016/j.biomaterials.2007.08.018
- Wang X, Xu S, Zhou S, et al. Topological design and additive manufacturing of porous metals for bone scaffolds and orthopaedic implants: a review. Biomaterials. 2016;83:127-141. doi: 10.1016/j.biomaterials.2016.01.012
- Chi P. Preparation and Performance Study of PCL/HA Spinal Interbody Fusion Cage Based on 3D Printing Technology. Beijing University of Chemical Technology, Beijing, CN. 2024. doi: 10.26939/d.cnki.gbhgu.2024.000660
- Fornes TD, Paul DR. Crystallization behavior of nylon 6 nanocomposites. Polymer. 2003;44(14):3945-3961. doi: 10.1016/s0032-3861(03)00344-6
- Chen DZ, Tang CY, Chan KC. et al. Dynamic mechanical properties and in vitro bioactivity of PHBHV/HA nanocomposite. Compos Sci Technol. 2007;67(7-8):1617-1626. doi: 10.1016/j.compscitech.2006.07.034
- Haq RHA, Rahman MNA, Arifin AMT, Hassan MF, Taib I, Wahit MU. Thermal properties of polycaprolactone (PCL) reinforced montmorillonite (MMT) and hydroxyapatite (HA) as an alternate of FDM composite filament. J Adv Res Fluid Mech Therm Sci. 2019;62(1), 112-121.
- Xiang S. 3D Printing Process and Performance Research of HA/PCL Bone Tissue Engineering Scaffold. Beijing University of Chemical Technology, Beijing, CN. 2020. doi: 10.26939/d.cnki.gbhgu.2019.000930
- Doyle SE, Henry L, McGennisken E. et al. Characterization of polycaprolactone nanohydroxyapatite composites with tunable degradability suitable for indirect printing. Polymers (Basel). 2021;13(2):295. doi: 10.3390/polym13020295
- Szcześ A, Hołysz L, Chibowski E. Synthesis of hydroxyapatite for biomedical applications. Adv Colloid Interface Sci. 2017;249:321-330. doi: 10.1016/j.cis.2017.04.007
- Gao J, Yu X, Wang X, He Y, Ding J. Biomaterial–related cell microenvironment in tissue engineering and regenerative medicine. Engineering. 2022;13:31-45. doi: 10.1016/j.eng.2021.11.025
- Díaz E, Sandonis I, Valle MB. In Vitro degradation of poly(caprolactone)/nHA composites. J Nanomater. 2014;2014:1-8. doi: 10.1155/2014/802435
- Díaz E, Sandonis I, Puerto I, Ibáñez M. In vitro degradation of PLLA/nHA composite scaffolds. Polym Eng Sci. 2014;54(11):2571-2578. doi: 10.1002/pen.23806
- Bartnikowski M, Dargaville TR, Ivanovski S, Hutmacher DW. Degradation mechanisms of polycaprolactone in the context of chemistry, geometry and environment. Prog Polym Sci. 2019;96:1-20. doi: 10.1016/j.progpolymsci.2019.05.004
- Casarin SA, Malmonge SM, Kobayashi M, Agnelli JM. Study on in vitro degradation of bioabsorbable polymers poly (hydroxybutyrate-co-valerate)-(PHBV) and poly (caprolactone)-(PCL). J Nanobiotechnol. 2011;2(3): 207-215. doi: 10.4236/jbnb.2011.23026
- Cho SJ, Jung SM, Kang M, Shin HS, Youk JH. Preparation of hydrophilic PCL nanofiber scaffolds via electrospinning of PCL/PVP-b-PCL block copolymers for enhanced cell biocompatibility. Polymer (Basel). 2015;69:95-102. doi: 10.1016/j.polymer.2015.05.037
- Jeong H, Rho J, Shin JY, Lee DY, Hwang T, Kim KJ. Mechanical properties and cytotoxicity of PLA/PCL films. Biomed Eng Lett. 2018;8(3):267-272. doi: 10.1007/s13534-018-0065-4