Development and characterization of graphene derivative–GelMA hybrid bioinks for the generation of bioartificial tissue substitutes via 3D bioprinting
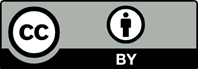
The fabrication of bioartificial tissue substitutes is a complex process that relies on the application of innovative biomaterials and manufacturing techniques enabling the generation of cell-laden scaffolds mimicking natural tissue interfaces. Among the many biomaterials, gelatin methacryloyl (GelMA) hydrogels have shown great potential for three-dimensional (3D) bioprinting-based tissue engineering due to their high biocompatibility, biodegradability, and tunable mechanical properties. In this study, the potential use of hybrid hydrogels based on GelMA and a highly purified graphene-derivative (BioGraph) as biomaterials bioinks for extrusion-based 3D bioprinting was investigated. Formulations containing BioGraph concentrations of up to 0.1% w/v were well-suited for this technique, showing good extrudability with reduced clogging at the printing temperatures, effective photocrosslinking at the irradiances tested, high shape fidelity, and high resolution of the printed scaffold. In situ photocrosslinking tests revealed that BioGraph concentration decreased the speed of the photocrosslinking and the stiffness of the cured matrix. In vitro studies indicated that BioGraph content ≤0.1% w/v did not have an adverse impact on the viability and proliferation of rat adipose-derived mesenchymal stem cells (r-AMSCs). Similarly, acellular scaffolds implanted subcutaneously in rats showed a local macrophage-mediated inflammatory reaction and a collagen encapsulation process without any affection of surrounded host tissues. The addition of lower concentrations of BioGraph (0.025% w/v) to the matrix resulted in enhanced macrophagic interactions and scaffold degradation in vivo, and r-AMSCs growth and proliferation in vitro. In conclusion, the GelMA–BioGraph hybrid hydrogels developed here demonstrate enhanced rheological and biological properties, tailored for extrusion-based 3D bioprinting with applications in the engineering of soft (neural, liver, etc.) or hard (bone) tissues.

- Timmis A, Townsend N, Gale CP, et al. European Society of Cardiology: Cardiovascular Disease Statistics 2019. Eur Heart J. Jan 1 2020;41(1):12-85. doi: 10.1093/eurheartj/ehz859
- Injury GBDTB, Spinal Cord Injury C. Global, regional, and national burden of traumatic brain injury and spinal cord injury, 1990-2016: a systematic analysis for the Global Burden of Disease Study 2016. Lancet Neurol. 2019;18(1):56-87. doi: 10.1016/S1474-4422(18)30415-0
- Tripathi S, Mandal SS, Bauri S, Maiti P. 3D bioprinting and its innovative approach for biomedical applications. MedComm (2020). 2023;4(1):e194. doi: 10.1002/mco2.194
- Zaszczynska A, Moczulska-Heljak M, Gradys A, Sajkiewicz P. Advances in 3D printing for tissue engineering. Materials (Basel). 2021;14(12):3149. doi: 10.3390/ma14123149
- Ng WL, Shkolnikov V. Jetting-based bioprinting: process, dispense physics, and applications. Bio-Des Manuf. 2024;7(5):771-799. doi: 10.1007/s42242-024-00285-3
- Li Y, Zhang X, Zhang X, Zhang Y, Hou D. Recent progress of the vat photopolymerization technique in tissue engineering: a brief review of mechanisms, methods, materials, and applications. Polymers (Basel). 2023;15(19):3940. doi: 10.3390/polym15193940
- Saini G, Segaran N, Mayer JL, Saini A, Albadawi H, Oklu R. Applications of 3D bioprinting in tissue engineering and regenerative medicine. J Clin Med. 2021;10(21):4966. doi: 10.3390/jcm10214966
- Liang K. Tissue bioprinting: promise and challenges. Bioengineering (Basel). 2023;10(12):1400. doi: 10.3390/bioengineering10121400
- Gomez-Florit M, Pardo A, Domingues RMA, et al. Natural-based hydrogels for tissue engineering applications. Molecules. 2020;25(24):5858. doi: 10.3390/molecules25245858
- Gyles DA, Castro LD, Silva JOC, Ribeiro-Costa RM. A review of the designs and prominent biomedical advances of natural and synthetic hydrogel formulations. Eur Polym J. 2017;88:373-392. doi: 10.1016/j.eurpolymj.2017.01.027
- García-García ÓD, Chato-Astrain J, Hochuli AHD, Pozzobon M, Carriel V. Decellularized tissue-derived materials for grafts development. In: Maia FRA, Oliveira JM, Reis RL. Handbook of the Extracellular Matrix. Cham: Springer; 2024:1011-1045:chap. 49. doi: 10.1007/978-3-030-92090-6_49-1
- Roche CD, Sharma P, Ashton AW, Jackson C, Xue M, Gentile C. Printability, durability, contractility and vascular network formation in 3D bioprinted cardiac endothelial cells using alginate-gelatin hydrogels. Front Bioeng Biotechnol. 2021;9:636257. doi: 10.3389/fbioe.2021.636257
- Moro A, Samanta S, Honkamaki L, et al. Hyaluronic acid based next generation bioink for 3D bioprinting of human stem cell derived corneal stromal model with innervation. Biofabrication. 2022;15(1):015020. doi: 10.1088/1758-5090/acab34
- Hewawasam RS, Blomberg R, Serbedzija P, Magin CM. Chemical modification of human decellularized extracellular matrix for incorporation into phototunable hybrid-hydrogel models of tissue fibrosis. ACS Appl Mater Interfaces. 2023;15(12):15071-15083. doi: 10.1021/acsami.2c18330
- Ndlovu SP, Ngece K, Alven S, Aderibigbe BA. Gelatin-based hybrid scaffolds: promising wound dressings. Polymers (Basel). 2021;13(17):2959. doi: 10.3390/polym13172959
- Lukin I, Erezuma I, Maeso L, et al. Progress in gelatin as biomaterial for tissue engineering. Pharmaceutics. 2022;14(6):1177. doi: 10.3390/pharmaceutics14061177
- Ribeiro AM, Meneses AC, Neumann IA. Polymeric nanoparticles and sponges in the control and stagnation of bleeding and wound healing. Desi Nanostruct Versatile Therap Appl. 2018;2018:189-219. doi: 10.1016/B978-0-12-813667-6.00005-X
- Wang X, Ao Q, Tian X, et al. Gelatin-Based Hydrogels for Organ 3D Bioprinting. Polymers (Basel). 2017; 9(9):401. doi: 10.3390/polym9090401
- Agten H, Van Hoven I, Van Hoorick J, Van Vlierberghe S, Luyten FP, Bloemen V. In vitro and in vivo evaluation of periosteum-derived cells and iPSC-derived chondrocytes encapsulated in GelMA for osteochondral tissue engineering. Front Bioeng Biotechnol. 2024; “12:1386692. doi: 10.3389/fbioe.2024.1386692
- Van Den Bulcke AI, Bogdanov B, De Rooze N, Schacht EH, Cornelissen M, Berghmans H. Structural and rheological properties of methacrylamide modified gelatin hydrogels. Biomacromolecules. 2000;1(1):31-38. doi: 10.1021/bm990017d
- Bedell ML, Torres AL, Hogan KJ, et al. Human gelatin-based composite hydrogels for osteochondral tissue engineering and their adaptation into bioinks for extrusion, inkjet, and digital light processing bioprinting. Biofabrication. 2022;14(4):10.1088/1758-5090/ac8768. doi: 10.1088/1758-5090/ac8768
- Lim KS, Klotz BJ, Lindberg GCJ, et al. Visible light cross-linking of gelatin hydrogels offers an enhanced cell microenvironment with improved light penetration depth. Macromol Biosci. 2019;19(6):e1900098. doi: 10.1002/mabi.201900098
- Koti P, Muselimyan N, Mirdamadi E, Asfour H, Sarvazyan NA. Use of GelMA for 3D printing of cardiac myocytes and fibroblasts. J 3D Print Med. 2019;3(1):11-22. doi: 10.2217/3dp-2018-0017
- Xu L, Zhang Z, Jorgensen AM, et al. Bioprinting a skin patch with dual-crosslinked gelatin (GelMA) and silk fibroin (SilMA): an approach to accelerating cutaneous wound healing. Mater Today Bio. 2023;18:100550. doi: 10.1016/j.mtbio.2023.100550
- Klotz BJ, Lim KS, Chang YX, et al. Engineering of a complex bone tissue model with endothelialised channels and capillary-like networks. Eur Cell Mater. 2018;35:335-348. doi: 10.22203/eCM.v035a23
- Hu C, Ahmad T, Haider MS, et al. A thermogelling organic-inorganic hybrid hydrogel with excellent printability, shape fidelity and cytocompatibility for 3D bioprinting. Biofabrication. 2022;14(2):025005. doi: 10.1088/1758-5090/ac40ee
- Liu S, Bernhardt A, Wirsig K, et al. Synergy of inorganic and organic inks in bioprinted tissue substitutes: Construct stability and cell response during long-term cultivation in vitro. Compos B: Eng. 2023;261:110804. doi: 10.1016/j.compositesb.2023.110804
- Fang X, Guo H, Zhang W, et al. Reduced graphene oxide- GelMA-PCL hybrid nanofibers for peripheral nerve regeneration. J Mater Chem B. 2020;8(46):10593-10601. doi: 10.1039/d0tb00779j
- Lopez-Gonzalez I, Zamora-Ledezma C, Sanchez-Lorencio MI, Tristante Barrenechea E, Gabaldon-Hernandez JA, Meseguer-Olmo L. Modifications in gene expression in the process of osteoblastic differentiation of multipotent bone marrow-derived human mesenchymal stem cells induced by a novel osteoinductive porous medical-grade 3D-printed poly(epsilon-caprolactone)/beta-tricalcium phosphate composite. Int J Mol Sci. 2021;22(20):11216. doi: 10.3390/ijms222011216
- Yaragalla S, Bhavitha KB, Athanassiou A. A review on graphene based materials and their antimicrobial properties. Coatings. 2021;11(10):1197. doi: 10.3390/coatings11101197
- Patil R, Alimperti S. Graphene in 3D bioprinting. J Funct Biomater. 2024;15(4):82. doi: 10.3390/jfb15040082
- Magne TM, de Oliveira Vieira T, Alencar LMR, et al. Graphene and its derivatives: understanding the main chemical and medicinal chemistry roles for biomedical applications. J Nanostructure Chem. 2022;12(5):693-727. doi: 10.1007/s40097-021-00444-3
- Kosowska K, Korycka P, Jankowska-Snopkiewicz K, et al. Graphene oxide (go)-based bioink with enhanced 3d printability and mechanical properties for tissue engineering applications. Nanomaterials (Basel). 2024;14(9):760. doi: 10.3390/nano14090760
- Vijayavenkataraman S, Thaharah S, Zhang S, Lu WF, Fuh JYH. 3D-printed PCL/rGO conductive scaffolds for peripheral nerve injury repair. Artif Organs. 2019;43(5):515-523. doi: 10.1111/aor.13360
- Magaz A, Li X, Gough JE, Blaker JJ. Graphene oxide and electroactive reduced graphene oxide-based composite fibrous scaffolds for engineering excitable nerve tissue. Mater Sci Eng C Mater Biol Appl. 2021;119:111632. doi: 10.1016/j.msec.2020.111632
- Saito N, Aoki K, Usui Y, et al. Application of carbon fibers to biomaterials: a new era of nano-level control of carbon fibers after 30-years of development. Chem Soc Rev. 2011;40(7):3824-3834. doi: 10.1039/c0cs00120a
- Mehrabi A, Baheiraei N, Adabi M, Amirkhani Z. Development of a novel electroactive cardiac patch based on carbon nanofibers and gelatin encouraging vascularization. Appl Biochem Biotechnol. 2020;190(3):931-948. doi: 10.1007/s12010-019-03135-6
- Salesa B, Assis M, Andres J, Serrano-Aroca A. Carbon nanofibers versus silver nanoparticles: time-dependent cytotoxicity, proliferation, and gene expression. Biomedicines. 2021;9(9):1155. doi: 10.3390/biomedicines9091155
- Rivera-Briso AL, Aachmann FL, Moreno-Manzano V, Serrano-Aroca A. Graphene oxide nanosheets versus carbon nanofibers: enhancement of physical and biological properties of poly(3-hydroxybutyrate-co-3- hydroxyvalerate) films for biomedical applications. Int J Biol Macromol. 2020;143:1000-1008. doi: 10.1016/j.ijbiomac.2019.10.034
- de Frutos S, Griera M, Lavín-Lopez MDP, et al. A new graphene-based nanomaterial increases lipolysis and reduces body weight gain through integrin linked kinase (ILK). Biomater Sci. 2023;11(14):4916-4929. doi: 10.1039/d2bm01791a
- Lavín-López MdP, Torres-Torresano M, García-Cuesta EM, et al. A graphene-based bioactive product with a non-immunological impact on mononuclear cell populations from healthy volunteers. Nanomaterials. 2024;14(23):1945. doi: 10.3390/nano14231945
- Etayo-Escanilla M, Campillo N, Avila-Fernandez P, et al. Comparison of printable biomaterials for use in neural tissue engineering: an in vitro characterization and in vivo biocompatibility assessment. Polymers (Basel). 2024;16(10):1426. doi: 10.3390/polym16101426
- Chato-Astrain J, Chato-Astrain I, Sanchez-Porras D, et al. Generation of a novel human dermal substitute functionalized with antibiotic-loaded nanostructured lipid carriers (NLCs) with antimicrobial properties for tissue engineering. J Nanobiotechnology. 2020;18(1):174. doi: 10.1186/s12951-020-00732-0
- Carriel V, Scionti G, Campos F, et al. In vitro characterization of a nanostructured fibrin agarose bio-artificial nerve substitute. J Tissue Eng Regen Med. 2017;11(5):1412-1426. doi: 10.1002/term.2039
- Gila-Vilchez C, Manas-Torres MC, Garcia-Garcia OD, et al. Biocompatible short-peptides fibrin co-assembled hydrogels. ACS Appl Polym Mater. 2023;5(3):2154-2165. doi: 10.1021/acsapm.2c02164
- Campos F, Bonhome-Espinosa AB, Vizcaino G, et al. Generation of genipin cross-linked fibrin-agarose hydrogel tissue-like models for tissue engineering applications. Biomed Mater. 2018;13(2):025021. doi: 10.1088/1748-605X/aa9ad2
- Chato-Astrain J, Campos F, Roda O, et al. In vivo evaluation of nanostructured fibrin-agarose hydrogels with mesenchymal stem cells for peripheral nerve repair. Front Cell Neurosci. 2018;12:501. doi: 10.3389/fncel.2018.00501
- Lotfy A, Salama M, Zahran F, Jones E, Badawy A, Sobh M. Characterization of mesenchymal stem cells derived from rat bone marrow and adipose tissue: a comparative study. Int J Stem Cells. 2014;7(2):135-142. doi: 10.15283/ijsc.2014.7.2.135
- Sun CK, Yen CH, Lin YC, et al. Autologous transplantation of adipose-derived mesenchymal stem cells markedly reduced acute ischemia-reperfusion lung injury in a rodent model. J Transl Med. 2011;9:118. doi: 10.1186/1479-5876-9-118
- Durand-Herrera D, Campos F, Jaimes-Parra BD, et al. Wharton’s jelly-derived mesenchymal cells as a new source for the generation of microtissues for tissue engineering applications. Histochem Cell Biol. 2018;150(4):379-393. doi: 10.1007/s00418-018-1685-6
- Sanchez-Porras D, Bermejo-Casares F, Carmona R, Weiss T, Campos F, Carriel V. Tissue fixation and processing for the histological identification of lipids. Methods Mol Biol. 2023;2566:175-186. doi: 10.1007/978-1-0716-2675-7_14
- Garcia-Garcia OD, El Soury M, Gonzalez-Quevedo D, et al. Histological, biomechanical, and biological properties of genipin-crosslinked decellularized peripheral nerves. Int J Mol Sci. 2021;22(2):674. doi: 10.3390/ijms22020674
- Geim AK, Novoselov KS. The rise of graphene. Nat Mater. 2007;6(3):183-191. doi: 10.1038/nmat1849
- Novoselov KS, Fal’ko VI, Colombo L, Gellert PR, Schwab MG, Kim K. A roadmap for graphene. Nature. 2012;490(7419):192-200. doi: 10.1038/nature11458
- Gu H, Tang H, Xiong P, Zhou Z. Biomarkers-based biosensing and bioimaging with graphene for cancer diagnosis. Nanomaterials (Basel). 2019;9(1):130. doi: 10.3390/nano9010130
- Li X, Chen A, Liu Y, Li L. Preparation and rectal administration of hydroxybutyl chitosan/graphene oxide composite thermosensitive hydrogel. React Funct Polym. 2023;189:105608. doi: 10.1016/j.reactfunctpolym.2023.105608
- Rajaei M, Rashedi H, Yazdian F, Navaei-Nigjeh M, Rahdar A, Díez-Pascual AM. Chitosan/agarose/graphene oxide nanohydrogel as drug delivery system of 5-fluorouracil in breast cancer therapy. J Drug Deliv Sci Technol. 2023;82:104307. doi: 10.1016/j.jddst.2023.104307
- Chen C, Xi Y, Weng Y. Progress in the development of graphene-based biomaterials for tissue engineering and regeneration. Materials (Basel). 2022;15(6):2164. doi: 10.3390/ma15062164
- de Carvalho JO, de Carvalho Oliveira F, Freitas SAP, et al. Carbon nanomaterials for treating osteoporotic vertebral fractures. Curr Osteoporos Rep. 2018;16(5):626-634. doi: 10.1007/s11914-018-0476-2
- Aparicio-Collado JL, Zheng Q, Molina-Mateo J, et al. Engineered highly porous polyvinyl alcohol hydrogels with poly(3-hydroxybutyrate-co-3-hydroxyvalerate) and graphene nanosheets for musculoskeletal tissue engineering: morphology, water sorption, thermal, mechanical, electrical properties, and biocompatibility. Materials (Basel). 2023;16(8):3114. doi: 10.3390/ma16083114
- Li A, Zhang C, Zhang YF. Thermal conductivity of graphene-polymer composites: mechanisms, properties, and applications. Polymers (Basel). 2017;9(9):437. doi: 10.3390/polym9090437
- Wu JB, Lin ML, Cong X, Liu HN, Tan PH. Raman spectroscopy of graphene-based materials and its applications in related devices. Chem Soc Rev. 2018;47(5):1822-1873. doi: 10.1039/c6cs00915h
- Sato Y, Shibata K, Kataoka H, et al. Strict preparation and evaluation of water-soluble hat-stacked carbon nanofibers for biomedical application and their high biocompatibility: influence of nanofiber-surface functional groups on cytotoxicity. Mol Biosyst. 2005;1(2):142-145. doi: 10.1039/b501222h
- Wang W, Junior JRP, Nalesso PRL, et al. Engineered 3D printed poly(varepsilon-caprolactone)/graphene scaffolds for bone tissue engineering. Mater Sci Eng C Mater Biol Appl. 2019;100:759-770. doi: 10.1016/j.msec.2019.03.047
- Ferreras A, Matesanz A, Mendizabal J, et al. Light-responsive and antibacterial graphenic materials as a holistic approach to tissue engineering. ACS Nanosci Au. 2024;4(4):263-272. doi: 10.1021/acsnanoscienceau.4c00006
- Rivera-Briso AL, Aparicio-Collado JL, Serra RSI, Serrano- Aroca A. Graphene oxide versus carbon nanofibers in poly(3-hydroxybutyrate-co-3-hydroxyvalerate) films: degradation in simulated intestinal environments. Polymers (Basel). 2022;14(2):348. doi: 10.3390/polym14020348
- Lee WC, Lim CH, Shi H, et al. Origin of enhanced stem cell growth and differentiation on graphene and graphene oxide. ACS Nano. 2011;5(9):7334-7341. doi: 10.1021/nn202190c
- Murjani BO, Kadu PS, Bansod M, Vaidya SS, Yadav MD. Carbon nanotubes in biomedical applications: current status, promises, and challenges. Carbon Lett. 2022;32(5):1207-1226. doi: 10.1007/s42823-022-00364-4
- Shahazi R, Majumdar S, Saddam AI, Mondal J, Rahman MM, Alam MM. Carbon nanomaterials for biomedical applications: a comprehensive review. Nano Carbons. 2023;1(1):448. doi: 10.59400/n-c.v1i1.448
- Kalman J, Merino C, Fernandez-Cruz ML, Navas JM. Usefulness of fish cell lines for the initial characterization of toxicity and cellular fate of graphene-related materials (carbon nanofibers and graphene oxide). Chemosphere. 2019;218:347-358. doi: 10.1016/j.chemosphere.2018.11.130
- Shin SR, Zihlmann C, Akbari M, et al. Reduced graphene oxide-GelMA hybrid hydrogels as scaffolds for cardiac tissue engineering. Small. 2016;12(27):3677-3689. doi: 10.1002/smll.201600178
- Wei Z, Harris BT, Zhang LG. Gelatin methacrylamide hydrogel with graphene nanoplatelets for neural cell-laden 3D bioprinting. Annu Int Conf IEEE Eng Med Biol Soc. 2016;2016:4185-4188. doi: 10.1109/EMBC.2016.7591649
- Flachs D, Etzel J, Mayer M, et al. Characterization of electrically conductive, printable ink based on alginate hydrogel and graphene nanoplatelets. Biomed Eng Adv. 2022;4:100045. doi: 10.1016/j.bea.2022.100045
- Huang H-Y, Fan F-Y, Shen Y-K, et al. 3D poly-ε-caprolactone/ graphene porous scaffolds for bone tissue engineering. Colloids Surf A: Physicochem Eng Aspects. 2020;606:125393. doi: 10.1016/j.colsurfa.2020.125393
- Guimarães CF, Gasperini L, Marques AP, Reis RL. The stiffness of living tissues and its implications for tissue engineering. Nat Rev Mater. 2020;5(5):351-370. doi: 10.1038/s41578-019-0169-1
- Ahmed J, Mulla M, Maniruzzaman M. Rheological and dielectric behavior of 3D-printable chitosan/graphene oxide hydrogels. ACS Biomater Sci Eng. 2020;6(1):88-99. doi: 10.1021/acsbiomaterials.9b00201
- Li H, Liu S, Li L. Rheological study on 3D printability of alginate hydrogel and effect of graphene oxide. Int J Bioprint. 2024;2(2):54-66. doi: 10.18063/ijb.2016.02.007
- Bao Q, Zhang H, Yang Jx, et al. Graphene–polymer nanofiber membrane for ultrafast photonics. Adv Funct Mater. 2010;20(5):782-791. doi: 10.1002/adfm.200901658
- Wang X, Zhi L, Mullen K. Transparent, conductive graphene electrodes for dye-sensitized solar cells. Nano Lett. 2008;8(1):323-327. doi: 10.1021/nl072838r
- Galano A. Carbon nanotubes as free-radical scavengers. J Phys Chem C. 2008;112(24):8922-8927. doi: 10.1021/jp801379g
- Engstrom TA, Pogoda K, Cruz K, Janmey PA, Schwarz JM. Compression stiffening in biological tissues: On the possibility of classic elasticity origins. Phys Rev E. 2019;99(5-1):052413. doi: 10.1103/PhysRevE.99.052413
- Mishra A, Cleveland RO. Rheological properties of porcine organs: measurements and fractional viscoelastic model. Front Bioeng Biotechnol. 2024;12:1386955. doi: 10.3389/fbioe.2024.1386955
- Biggs LC, Kim CS, Miroshnikova YA, Wickstrom SA. Mechanical forces in the skin: roles in tissue architecture, stability, and function. J Invest Dermatol. 2020;140(2):284-290. doi: 10.1016/j.jid.2019.06.137
- Ma R, Wang Y, Qi H, et al. Nanocomposite sponges of sodium alginate/graphene oxide/polyvinyl alcohol as potential wound dressing: In vitro and in vivo evaluation. Compos Part B: Eng. 2019;167:396-405. doi: 10.1016/j.compositesb.2019.03.006
- Xu HQ, Liu JC, Zhang ZY, Xu CX. A review on cell damage, viability, and functionality during 3D bioprinting. Mil Med Res. 2022;9(1):70. doi: 10.1186/s40779-022-00429-5
- Middleton JC, Tipton AJ. Synthetic biodegradable polymers as orthopedic devices. Biomaterials. 2000;21(23): 2335-2346. doi: 10.1016/s0142-9612(00)00101-0
- Fournier E, Passirani C, Montero-Menei CN, Benoit JP. Biocompatibility of implantable synthetic polymeric drug carriers: focus on brain biocompatibility. Biomaterials. 2003;24(19):3311-3331. doi: 10.1016/s0142-9612(03)00161-3
- Nyska A, Schiffenbauer YS, Brami CT, Maronpot RR, Ramot Y. Histopathology of biodegradable polymers: challenges in interpretation and the use of a novel compact MRI for biocompatibility evaluation. Polym Adv Technol. 2014;25(5):461-467. doi: 10.1002/pat.3238
- Monteiro N, Thrivikraman G, Athirasala A, et al. Photopolymerization of cell-laden gelatin methacryloyl hydrogels using a dental curing light for regenerative dentistry. Dent Mater. 2018;34(3):389-399. doi: 10.1016/j.dental.2017.11.020
- Ding X, Pang Y, Liu Q, et al. GO-PEG represses the progression of liver inflammation via regulating the M1/M2 polarization of Kupffer cells. Small. 2024;20(26):e2306483. doi: 10.1002/smll.202306483
- Huaux F, d’Ursel de Bousies V, Parent MA, et al. Mesothelioma response to carbon nanotubes is associated with an early and selective accumulation of immunosuppressive monocytic cells. Part Fibre Toxicol. 2016;13(1):46. doi: 10.1186/s12989-016-0158-0
- Zhang X, Luo M, Zhang J, et al. Carbon nanotubes promote alveolar macrophages toward M2 polarization mediated epithelial-mesenchymal transition and fibroblast-to-myofibroblast transdifferentiation. Nanotoxicology. 2021;15(5):588-604. doi: 10.1080/17435390.2021.1905098
- Zarrintaj P, Manouchehri S, Ahmadi Z, et al. Agarose-based biomaterials for tissue engineering. Carbohydr Polym. 2018;187:66-84. doi: 10.1016/j.carbpol.2018.01.060
- Koffler J, Zhu W, Qu X, et al. Biomimetic 3D-printed scaffolds for spinal cord injury repair. Nat Med. 2019;25(2): 263-269. doi: 10.1038/s41591-018-0296-z