3D bioprinting and label-free imaging: Bridging innovations for organoid research
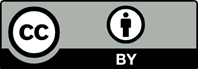
Organoids are three-dimensional (3D) and multicellular structures that more closely mimic the architecture and functions of human organs. These in vitro systems are derived from pluripotent stem cells, tissue-resident stem cells, or organ-specific progenitors. Despite their potential, conventional organoid development methods are limited by inconsistencies in formation and the absence of complete microenvironmental cues, which reduce reproducibility in larger organ models. In contrast, 3D bioprinting techniques offer a precise layer-by-layer construction approach that enables superior spatial control, scalability, and uniformity in organoid formation. In this review, we examine the principles, strengths, applications, and limitations of these imaging methods, offering insights into their potential to drive further innovations in the rapidly evolving field of organoid imaging. To track the dynamic processes of cell growth, differentiation, and organization during organoid development and maturation, advanced imaging technologies are crucial. Traditional optical imaging methods, however, require exogenous labeling agents to enhance contrast, which can damage samples through photobleaching and phototoxicity. Label-free and real-time imaging modalities, by contrast, offer non-invasive and non-destructive monitoring of organoids, preserving sample integrity and enabling longitudinal studies. This review highlights the benefits of bioprinting technologies in overcoming current limitations in organoid development and provides a comprehensive overview of label-free and real-time imaging technologies for organoids. In this review, we examine the principles, strengths, applications, and limitations of these imaging methods, offering insights into their potential to drive further innovations in the rapidly evolving field of organoid imaging.

- Zhao Z, Chen X, Dowbaj A, et al. Organoids. Nat Rev Methods Primers. 2022;2(1):94. doi: 10.1038/s43586-022-00174-y
- Lancaster M, Renner M, Martin CA, et al. Cerebral organoids model human brain development and microcephaly. Nature. 2013;501(7467):373-379. doi: 10.1038/nature12517
- Hofer M, Lutolf M. Engineering organoids. Nat Rev Mater. 2021;6(5):402-420. doi: 10.1038/s41578-021-00279-y
- Paşca SP. Assembling human brain organoids. Science. 2019;363(6423):126-127. doi: 10.1126/science.aau5729
- Lullo E, Kriegstein AR. The use of brain organoids to investigate neural development and disease. Nat Rev Neurosci. 2017;18(10):573-584. doi: 10.1038/nrn.2017.107
- Puschhof J, Pleguezuelos-Manzano C, Martinez-Silgado A, et al. Intestinal organoid cocultures with microbes. Nat Protoc. 2021;16(10):4633-4649. doi: 10.1038/s41596-021-00589-z
- Harrison S, Baumgarten S, Verma R, Lunov O, Dejneka A, Sullivan GJ. Liver organoids: recent developments, limitations and potential. Front Med. 2021;8:574047. doi: 10.3389/fmed.2021.574047
- Takasato M, Er PX, Chiu HS, Little MH. Generation of kidney organoids from human pluripotent stem cells. Nat Protoc. 2016;11(9):1681-1692. doi: 10.1038/nprot.2016.098
- Lewis-Israeli Y, Wasserman A, Gabalski M, et al. Self-assembling human heart organoids for the modeling of cardiac development and congenital heart disease. Nat Commun. 2021;12(1):5142. doi: 10.1038/s41467-021-25329-5
- Ogundipe V, Groen A, Hosper N, et al. Generation and differentiation of adult tissue-derived human thyroid organoids. Stem Cell Reports. 2021;16(4):913-925. doi: 10.1016/j.stemcr.2021.02.011
- Völkner M, Zschätzsch M, Rostovskaya M, et al. Retinal organoids from pluripotent stem cells efficiently recapitulate retinogenesis. Stem Cell Reports. 2016;6(4):525-538. doi: 10.1016/j.stemcr.2016.03.001
- Fernández P, Buchmann B, Goychuk A, et al. Surface-tension-induced budding drives alveologenesis in human mammary gland organoids. Nat Phys. 2021;17(10):1130-1136. doi: 10.1038/s41567-021-01336-7
- Perrone F, Zilbauer M. Biobanking of human gut organoids for translational research. Exp Mol Med. 2021;53(10):1451-1458. doi: 10.1038/s12276-021-00606-x
- Miller A, Dye B, Ferrer-Torres D, et al. Generation of lung organoids from human pluripotent stem cells in vitro. Nat Protoc. 2019;14(2):518-540. doi: 10.1038/s41596-018-0104-8
- Murphy S, Atala A. 3D bioprinting of tissues and organs. Nat Biotechnol. 2014;32(8):773-785. doi: 10.1038/nbt.2958
- Kolesky D, Truby R, Gladman A, et al. 3D bioprinting of vascularized, heterogeneous cell‐laden tissue constructs. Adv Materi. 2014;26(19):3124-3130. doi: 10.1002/adma.201305506
- Lawlor K, Vanslambrouck J, Higgins J, et al. Cellular extrusion bioprinting improves kidney organoid reproducibility and conformation. Nat Mater. 2021;20(2):260-271. doi: 10.1038/s41563-020-00853-9
- Lee A, Hudson A, Shiwarski D, et al. 3D bioprinting of collagen to rebuild components of the human heart. Science. 2019;365(6452):482-487. doi: 10.1126/science.aav9051
- Rios A, Clevers H. Imaging organoids: a bright future ahead. Nat Methods. 2018;15(1):24-26. doi: 10.1038/nmeth.4537
- Beghin A, Grenci G, Sahni G, et al. Automated high-speed 3D imaging of organoid cultures with multi-scale phenotypic quantification. Nat Methods. 2022;19(7):881-892. doi: 10.1038/s41592-022-01508-0
- Okkelman I, Foley T, Papkovsky D, et al. Live cell imaging of mouse intestinal organoids reveals heterogeneity in their oxygenation. Biomaterials. 2017;146:86-96. doi: 10.1016/j.biomaterials.2017.08.043
- Keshara R, Kim Y, Grapin-Botton A. Organoid imaging: seeing development and function. Annu Rev Cell Dev Biol. 2022;38(1):447-466. doi: 10.1146/annurev-cellbio-120320-035146
- Medeiros G, Ortiz R, Strnad P, et al. Multiscale light-sheet organoid imaging framework. Nat Commun. 2022;13(1): 4864. doi: 10.1038/s41467-022-32465-z
- Dmitriev R, Okkelman I. Multi-parameter fluorescence lifetime imaging microscopy (FLIM) for imaging metabolism in the intestinal Organoids model. Biophys J. 2020;118(3): 330a. doi: 10.1016/j.bpj.2019.11.1846
- Dekkers J, Alieva M, Wellens L, et al. High-resolution 3D imaging of fixed and cleared organoids. Nat Protoc. 2019;14(6):1756-1771. doi: 10.1038/s41596-019-0160-8
- Chen Y, Chauhan S, Gong C, et al. Low-cost and scalable projected light-sheet microscopy for the high-resolution imaging of cleared tissue and living samples. Nat Biomed Eng. 2024;8:1109-1123. doi: 10.1038/s41551-024-01249-9
- Shaked N, Boppart S, Wang L, et al. Label-free biomedical optical imaging. Nat Photonics. 2023;17(12):1031-1041. doi: 10.1038/s41566-023-01299-6
- Stanly T, Suman R, Rani G, O’Toole PJ, Kaye P, Hitchcock IS. Quantitative optical diffraction tomography imaging of mouse platelets. Front Physiol. 2020;11:568087. doi: 10.3389/fphys.2020.568087
- Bouma B, Boer J, Huang D, et al. Optical coherence tomography. Nat Rev Methods Primers. 2022;2(1):79. doi: 10.1038/s43586-022-00162-2
- Barulin A, Park H, Park B, Kim I. Dual-wavelength UV-visible metalens for multispectral photoacoustic microscopy: A simulation study. Photoacoustics. 2023;32: 100545. doi: 10.1016/j.pacs.2023.100545
- Park B, Han M, Kim H, et al. Shear‐force photoacoustic microscopy: toward super‐resolution near‐field imaging. Laser Photonics Rev. 2022;16(12):2200296. doi: 10.1002/lpor.202200296
- Kim J, Heo D, Cho S, et al. Enhanced dual-mode imaging: superior photoacoustic and ultrasound microendoscopy in live pigs using a transparent ultrasound transducer. Sci Adv. 2024;10(47):eadq9960. doi: 10.1126/sciadv.adq9960
- Choi W, Park E, Jeon S, et al. Three-dimensional multistructural quantitative photoacoustic and US imaging of human feet in vivo. Radiology. 2022;303(2):467-473. doi: 10.1148/radiol.211029
- Ahn J, Baik J, Kim D, et al. In vivo photoacoustic monitoring of vasoconstriction induced by acute hyperglycemia. Photoacoustics. 2023;30:100485. doi: 10.1016/j.pacs.2023.100485
- Ahn J, Baik W, Kim Y, et al. Fully integrated photoacoustic microscopy and photoplethysmography of human in vivo. Photoacoustics. 2022;27:100374. doi: 10.1016/j.pacs.2022.100374
- Sivitilli A, Gosio J, Ghoshal B, et al. Robust production of uniform human cerebral organoids from pluripotent stem cells. Life Sci Alliance. 2020;3(5):202000707. doi: 10.26508/lsa.202000707
- Yang F, Bevilacqua C, Hambura S, et al. Pulsed stimulated brillouin microscopy enables high-sensitivity mechanical imaging of live and fragile biological specimens. Nat Methods. 2023;20(12):1971-1979. doi: 10.1038/s41592-023-02054-z
- Liang W, Chen D, Guan H, et al. Label-free metabolic imaging in vivo by two-photon fluorescence lifetime endomicroscopy. ACS Photonics. 2022;9(12):4017-4029. doi: 10.1021/acsphotonics.2c01493
- Scholler J, Groux K, Goureau O, et al. Dynamic full-field optical coherence tomography: 3D live-imaging of retinal organoids. Light Sci Appl. 2020;9(1):140. doi: 10.1038/s41377-020-00375-8
- Englert L, Lacalle‐Aurioles M, Mohamed N, et al. Fast 3D optoacoustic mesoscopy of neuromelanin through entire human midbrain organoids at single‐cell resolution. Laser Photonics Rev. 2023;17(8):2300443. doi: 10.1002/lpor.202300443
- Xue Y, Browne A, Tang W, et al. Retinal organoids long-term functional characterization using two-photon fluorescence lifetime and hyperspectral microscopy. Front Cell Neurosci. 2021;15:796903. doi: 10.3389/fncel.2021.796903
- Lee M, Lee J, Ha J, et al. Long-term three-dimensional high-resolution imaging of live unlabeled small intestinal organoids via low-coherence holotomography. Exp Mol Med. 2024;56:2162-2170. doi: 10.1038/s12276-024-01312-0
- Quadrato G, Brown J, Arlotta P. The promises and challenges of human brain organoids as models of neuropsychiatric disease. Nat Med. 2016;22(11):1220-1228. doi: 10.1038/nm.4214
- Velasco S, Kedaigle AJ, Simmons SK, et al. Individual brain organoids reproducibly form cell diversity of the human cerebral cortex. Nature. 2019;570(7762):523-527. doi: 10.1038/s41586-019-1289-x
- Hughes C, Postovit L, Lajoie G. Matrigel: a complex protein mixture required for optimal growth of cell culture. Proteomics. 2010;10(9):1886-1890. doi: 10.1002/pmic.200900758
- Benton G, Arnaoutova I, George J, Kleinman H, Koblinski J. Matrigel: from discovery and ECM mimicry to assays and models for cancer research. Adv Drug Deliv Rev. 2014;79:3-18. doi: 10.1016/j.addr.2014.06.005
- Gjorevski N, Lutolf M. Synthesis and characterization of well-defined hydrogel matrices and their application to intestinal stem cell and organoid culture. Nat Protoc. 2017;12(11):2263-2274. doi: 10.1038/nprot.2017.095
- Cleaver O, Melton D. Endothelial signaling during development. Nat Med. 2003;9(6):661-668. doi: 10.1038/nm0603-661
- Grebenyuk S, Ranga A. Engineering organoid vascularization. Front Bioeng Biotechnol. 2019;7:39. doi: 10.3389/fbioe.2019.00039
- Cakir B, Xiang Y, Tanaka Y, et al. Engineering of human brain organoids with a functional vascular-like system. Nat Methods. 2019;16(11):1169-1175. doi: 10.1038/s41592-019-0586-5
- Huch M, Dorrell C, Boj SF, et al. In vitro expansion of single Lgr5+ liver stem cells induced by Wnt-driven regeneration. Nature. 2013;494(7436):247-250. doi: 10.1038/nature11826
- Prior N, Inacio P, Huch M. Liver organoids: from basic research to therapeutic applications. Gut. 2019;68(12):2228-2237. doi: 10.1136/gutjnl-2019-319256
- Czerniecki S, Cruz N, Harder J, et al. High-throughput screening enhances kidney organoid differentiation from human pluripotent stem cells and enables automated multidimensional phenotyping. Cell stem cell. 2018;22(6): 929-940.e4. doi: 10.1016/j.stem.2018.04.022
- Combes A, Zappia L, Er P, Oshlack A, Little M. Single-cell analysis reveals congruence between kidney organoids and human fetal kidney. Genome Med. 2019;11:1-15. doi: 10.1186/s13073-019-0615-0
- Sato T, Clevers H. Growing self-organizing mini-guts from a single intestinal stem cell: mechanism and applications. Science. 2013;340(6137):1190-1194. doi: 10.1126/science.1234852
- Sachs N, Papaspyropoulos A, Zomer‐van Ommen D, et al. Long‐term expanding human airway organoids for disease modeling. EMBO J. 2019;38(4):e100300. doi: 10.15252/embj.2018100300
- Zhang Y, Haghiashtiani G, Hübscher T, et al. 3D extrusion bioprinting. Nat Rev Methods Primers. 2021;1(1):75. doi: 10.1038/s43586-021-00073-8
- Ramesh S, Harrysson O, Rao P, et al. Extrusion bioprinting: recent progress, challenges, and future opportunities. Bioprinting. 2021;21:e00116. doi: 10.1016/j.bprint.2020.e00116
- Ng L, Shkolnikov V. Jetting-based bioprinting: process, dispense physics, and applications. Bio-Design Manufactur. 2024;7(5):771-799. doi: 10.1007/s42242-024-00285-3
- Levato R, Dudaryeva O, Garciamendez-Mijares CE, et al. Light-based vat-polymerization bioprinting. Nat Rev Methods Primers. 2023;3(1):47. doi: 10.1038/s43586-023-00231-0
- Mohammadrezaei D, Podina L, De Silva J, Kohandel M. Cell viability prediction and optimization in extrusion-based bioprinting via neural network-based Bayesian optimization models. Biofabrication. 2024;16(2):025016. doi: 10.1088/1758-5090/ad17cf
- Xu T, Zhao W, Zhu J, Albanna MZ, Yoo J, Atala A. Complex heterogeneous tissue constructs containing multiple cell types prepared by inkjet printing technology. Biomaterials. 2013;34(1):130-139. doi: 10.1016/j.biomaterials.2012.09.035
- Li X, Liu B, Pei B, et al. Inkjet bioprinting of biomaterials. Chem Rev. 2020;120(19):10793-10833. doi: 10.1021/acs.chemrev.0c00008
- Keriquel V, Oliveira H, Rémy M, et al. In situ printing of mesenchymal stromal cells, by laser-assisted bioprinting, for in vivo bone regeneration applications. Sci Rep. 2017; 7(1):1778. doi: 10.1038/s41598-017-01914-x
- Koch L, Deiwick A, Schlie S, et al. Skin tissue generation by laser cell printing. Biotechnol Bioeng. 2012;109(7):1855-1863. doi: 10.1002/bit.24455
- Kérourédan O, Hakobyan D, Rémy M, et al. In situ prevascularization designed by laser-assisted bioprinting: effect on bone regeneration. Biofabrication. 2019;11(4):045002. doi: 10.1088/1758-5090/ab2620
- Chartrain N, Williams C, Whittington A. A review on fabricating tissue scaffolds using vat photopolymerization. Acta Biomater. 2018;74:90-111. doi: 10.1016/j.actbio.2018.05.010
- Li W, Mille L, Robledo J, Uribe T, Huerta V, Zhang Y. Recent advances in formulating and processing biomaterial inks for vat polymerization‐based 3D printing. Adv Healthc Mater. 2020;9(15):2000156. doi: 10.1002/adhm.202000156
- Jakab K, Norotte C, Marga F, Murphy K, Vunjak-Novakovic G, Forgacs G. Tissue engineering by self-assembly and bio-printing of living cells. Biofabrication. 2010;2(2): 022001. doi: 10.1088/1758-5082/2/2/022001
- Ning L, Chen X. A brief review of extrusion‐based tissue scaffold bio‐printing. Biotechnol J. 2017;12(8):1600671. doi: 10.1002/biot.201600671
- Nair K, Gandhi M, Khalil S, et al. Characterization of cell viability during bioprinting processes. Biotechnol J. 2009;4(8):1168-77. doi: 10.1002/biot.200900004
- Gao Q, He Y, Fu J, Liu A, Ma L. Coaxial nozzle-assisted 3D bioprinting with built-in microchannels for nutrients delivery. Biomaterials. 2015;61:203-215. doi: 10.1016/j.biomaterials.2015.05.031
- Malda J, Visser J, Melchels F, et al. 25th anniversary article: engineering hydrogels for biofabrication. Adv Mater. 2013;25(36):5011-5028. doi: 10.1002/adma.201302042
- Xu T, Jin J, Gregory C, Hickman J, Boland T. Inkjet printing of viable mammalian cells. Biomaterials. 2005;26(1):93-99. doi: 10.1016/j.biomaterials.2004.04.011
- Cui X, Boland T, Lima D, K. Lotz M. Thermal inkjet printing in tissue engineering and regenerative medicine. Recent Pat Drug Deliv Formul. 2012;6(2):149-155. doi: 10.2174/187221112800672949
- Derby B. Bioprinting: inkjet printing proteins and hybrid cell-containing materials and structures. J Mater Chem. 2008;18(47):5717-5721. doi: 10.1039/B807560C
- Saunders R, Derby B. Inkjet printing biomaterials for tissue engineering: bioprinting. Int Mater Rev. 2014;59(8):430-448. doi: 10.1179/1743280414Y.0000000040
- Lee W, Debasitis J, Lee VK, et al. Multi-layered culture of human skin fibroblasts and keratinocytes through three-dimensional freeform fabrication. Biomaterials. 2009;30(8):1587-1595. doi: 10.1016/j.biomaterials.2008.12.009
- Horváth L, Umehara Y, Jud C, Blank F, Petri-Fink A, Rothen- Rutishauser B. Engineering an in vitro air-blood barrier by 3D bioprinting. Sci Rep. 2015;5(1):7974. doi: 10.1038/srep07974
- Hospodiuk M, Dey M, Sosnoski D, Ozbolat IT. The bioink: a comprehensive review on bioprintable materials. Biotechnol Adv. 2017;35(2):217-239. doi: 10.1016/j.biotechadv.2016.12.006
- Ozbolat I, Yu Y. Bioprinting toward organ fabrication: challenges and future trends. IEEE Trans Biomed Eng. 2013; 60(3):691-699. doi: 10.1109/TBME.2013.2243912
- Liu W, Zhong Z, Hu N, et al. Coaxial extrusion bioprinting of 3D microfibrous constructs with cell-favorable gelatin methacryloyl microenvironments. Biofabrication. 2018; 10(2):024102. doi: 10.1088/1758-5090/aa9d44
- Melchels P, Feijen J, Grijpma D. A review on stereolithography and its applications in biomedical engineering. Biomaterials. 2010;31(24):6121-6130. doi: 10.1016/j.biomaterials.2010.04.050
- Chimene D, Lennox K, Kaunas R, Gaharwar A. Advanced bioinks for 3D printing: a materials science perspective. Ann Biomed Eng. 2016;44:2090-2102. doi: 10.1007/s10439-016-1638-y
- Lim K, Levato R, Costa P, et al. Bio-resin for high resolution lithography-based biofabrication of complex cell-laden constructs. Biofabrication. 2018;10(3):034101. doi: 10.1088/1758-5090/aac00c
- Tumbleston J, Shirvanyants D, Ermoshkin N, et al. Continuous liquid interface production of 3D objects. Science. 2015;347(6228):1349-1352. doi: 10.1126/science.aaa2397
- Grigoryan B, Paulsen S, Corbett D, et al. Multivascular networks and functional intravascular topologies within biocompatible hydrogels. Science. 2019;364(6439):458-464. doi: 10.1126/science.aav9750
- Sharifi S, Sharifi H, Akbari A, Chodosh J. Systematic optimization of visible light-induced crosslinking conditions of gelatin methacryloyl (GelMA). Sci Rep. 2021;11:23276. doi: 110.1038/s41598-021-02830-x
- Williams C, Malik A, Kim T, Manson P, Elisseeff J. Variable cytocompatibility of six cell lines with photoinitiators used for polymerizing hydrogels and cell encapsulation. Biomaterials. 2005;26(11):1211-1218. doi: 10.1016/j.biomaterials.2004.04.024
- Fairbanks B, Schwartz M, Bowman C, Anseth K. Photoinitiated polymerization of PEG-diacrylate with lithium phenyl-2, 4, 6-trimethylbenzoylphosphinate: polymerization rate and cytocompatibility. Biomaterials. 2009;30(35):6702-6707. doi: 10.1016/j.biomaterials.2009.08.055
- Yue K, Trujillo-de Santiago G, Alvarez MM, Tamayol A, Annabi N, Khademhosseini A. Synthesis, properties, and biomedical applications of gelatin methacryloyl (GelMA) hydrogels. Biomaterials. 2015;73:254-71. doi: 10.1016/j.biomaterials.2015.08.045
- Reid J, Mollica P, Bruno R, Sachs P. Consistent and reproducible cultures of large-scale 3D mammary epithelial structures using an accessible bioprinting platform. Breast Cancer Res. 2018;20:1-13. doi: 10.1186/s13058-018-1045-4
- Noor N, Shapira A, Edri R, Gal I, Wertheim L, Dvir T. 3D printing of personalized thick and perfusable cardiac patches and hearts. Adv Sci. 2019;6(11):1900344. doi: 10.1002/advs.201900344
- Pham M, Pollock K, Rose M, et al. Generation of human vascularized brain organoids. Neuroreport. 2018;29(7):588-593. doi: 10.1097/WNR.0000000000001014
- Lee J, Cuddihy M, Kotov N. Three-dimensional cell culture matrices: state of the art. Tissue Eng Part B Rev. 2008;14(1):61-86. doi: 10.1089/teb.2007.0150
- Caliari S, Burdick J. A practical guide to hydrogels for cell culture. Nat Methods. 2016;13(5):405-414. doi: 10.1038/nmeth.3839
- Heinrich M, Bansal R, Lammers T, Zhang Y, Michel Schiffelers R, Prakash J. 3D‐bioprinted mini‐brain: a glioblastoma model to study cellular interactions and therapeutics. Adv Mater. 2019;31(14):1806590. doi: 10.1002/adma.201806590
- Kratochvil M, Seymour A, Li TL, Paşca S, Kuo C, Heilshorn S. Engineered materials for organoid systems. Nat Rev Mater. 2019;4(9):606-622. doi: 10.1038/s41578-019-0129-9
- Wang H, Yu H, Zhou X, et al. An overview of extracellular matrix-based bioinks for 3D bioprinting. Front Bioeng Biotechnol. 2022;10:905438. doi: 10.3389/fbioe.2022.905438
- Bülow A, Schäfer B, Beier J. Three-dimensional bioprinting in soft tissue engineering for plastic and reconstructive surgery. Bioengineering. 2023;10(10):1232. doi: 10.3390/bioengineering10101232
- Andersen J, Revah O, Miura Y, et al. Generation of functional human 3D cortico-motor assembloids. Cell. 2020;183(7):1913-1929.e26. doi: 10.1016/j.cell.2020.11.017
- Qian X, Jacob F, Song M, Nguyen H, Song H, Ming Gl. Generation of human brain region–specific organoids using a miniaturized spinning bioreactor. Nat Protoc. 2018;13(3):565-580. doi: 10.1038/nprot.2017.152
- Wolf K, Weiss J, Uzel S, Skylar-Scott M, Lewis J. Biomanufacturing human tissues via organ building blocks. Cell Stem Cell. 2022;29(5):667-677. doi: 10.1016/j.stem.2022.04.012
- Mironov V, Mironov V. Organ printing: tissue spheroids as building blocks. Gene Cell. 2010;5(3):41. doi: 10.23868/gc121746
- Bagley J, Reumann D, Bian S, Lévi-Strauss J, Knoblich JA. Fused cerebral organoids model interactions between brain regions. Nat Methods. 2017;14(7):743-751. doi: 10.1038/nmeth.4304
- Lancaster M, Knoblich J. Generation of cerebral organoids from human pluripotent stem cells. Nat Protoc. 2014;9(10):2329-2340. doi: 10.1038/nprot.2014.158
- Driehuis E, Kretzschmar K, Clevers H. Establishment of patient-derived cancer organoids for drug-screening applications. Nat Protoc. 2020;15(10):3380-3409. doi: 10.1038/s41596-020-0379-4
- Heydari Z, Moeinvaziri F, Agarwal T, et al. Organoids: a novel modality in disease modeling. Biodes Manuf. 2021;4: 689-716. doi: 10.1007/s42242-021-00150-7
- Grassi L, Alfonsi R, Francescangeli F, et al. Organoids as a new model for improving regenerative medicine and cancer personalized therapy in renal diseases. Cell Death Dis. 2019;10(3):201. doi: 10.1038/s41419-019-1453-0
- Maharjan S, Ma C, Singh B, et al. Advanced 3D imaging and organoid bioprinting for biomedical research and therapeutic applications. Adv Drug Deliv Rev. 2024:115237. doi: 10.1016/j.addr.2024.115237
- Langer M, Cloetens P, Peyrin F. Regularization of phase retrieval with phase-attenuation duality prior for 3-D holotomography. IEEE Trans Image Process. 2010;19(9):2428-2436. doi: 10.1109/TIP.2010.2048608
- Jo J, Baek D, Hugonnet H, et al. 3D Measurements and characterizations of refractive index distributions of volume holographic gratings using low‐coherence holotomography. Adv Opt Mate. 2024;12(13):2302048. doi: 10.1002/adom.202302048
- Salucci S, Battistelli M, Burattini S, Sbrana F, Falcieri E. Holotomographic microscopy: a new approach to detect apoptotic cell features. Microsc Res Tech. 2020;83(12): 1464-1470. doi: 10.1002/jemt.23539
- Kim G, Hugonnet H, Kim K, et al. Holotomography. Nat Rev Methods Primers. 2024;4(1):51 doi: 10.1038/s43586-024-00327-1
- Park D, Lee D, Kim Y, et al. Cryobiopsy: a breakthrough strategy for clinical utilization of lung cancer organoids. Cells. 2023;12(14):1854. doi: 10.3390/cells12141854
- Ziemczonok M, Desissaire S, Neri J, et al. Tailored 3D microphantoms: an essential tool for quantitative phase tomography analysis of organoids. arXiv preprint arXiv: 240916888. 2024; doi: 10.48550/arXiv.2409.16888
- Kim D, Oh N, Kim K, et al. Label-free high-resolution 3D imaging of gold nanoparticles inside live cells using optical diffraction tomography. Methods. 2018;136:160-167. doi: 10.1016/j.ymeth.2017.07.008
- Kim K, Lee S, Yoon J, Heo J, Choi C, Park Y. Three-dimensional label-free imaging and quantification of lipid droplets in live hepatocytes. Sci Rep. 2016;6(1):36815. doi: 10.1038/srep36815
- Gao W, Wu X. Differences between time domain and Fourier domain optical coherence tomography in imaging tissues. J Microsc. 2017;268(2):119-128. doi: 10.1111/jmi.12592
- Morishita R, Suzuki T, Mukherjee P, et al. Label-free intratissue activity imaging of alveolar organoids with dynamic optical coherence tomography. Biomed Opt Express. 2023;14(5):2333-2351. doi: 10.1364/BOE.488097
- Gil D, Deming D, Skala M. Volumetric growth tracking of patient-derived cancer organoids using optical coherence tomography. Biomed Opt Express. 2021;12(7):3789-3805. doi: 10.1364/BOE.428197
- Bao D, Wang L, Zhou X, Yang S, He K, Xu M. Automated detection and growth tracking of 3D bio-printed organoid clusters using optical coherence tomography with deep convolutional neural networks. Front Bioeng Biotechnol. 2023;11:1133090. doi: 10.3389/fbioe.2023.1133090
- Dubois A, Vabre L, Boccara A, Beaurepaire E. High-resolution full-field optical coherence tomography with a Linnik microscope. Appl Opt. 2002;41(4):805-812. doi: 10.1364/ao.41.000805
- Hosseinaee Z, Tummon Simmons J, Reza P. Dual-modal photoacoustic imaging and optical coherence tomography. Front Phys. 2021;8:616618. doi: 10.3389/fphy.2020.616618
- Park B, Park S, Kim J, Kim C. Listening to drug delivery and responses via photoacoustic imaging. Adv Drug Deliv Rev. 2022;184:114235. doi: 10.1016/j.addr.2022.114235
- Cho S, Kim M, Ahn J, et al. An ultrasensitive and broadband transparent ultrasound transducer for ultrasound and photoacoustic imaging in-vivo. Nat Commun. 2024;15(1):1444. doi: 10.1038/s41467-024-45273-4
- Yoon C, Park E, Misra S, et al. Deep learning-based virtual staining, segmentation, and classification in label-free photoacoustic histology of human specimens. Light Sci Appl. 2024;13(1):226. doi: 10.1038/s41377-024-01554-7
- Park J, Choi S, Knieling F, et al. Clinical translation of photoacoustic imaging. Nat Rev Bioeng. 2024:1-20. doi: 10.1038/s44222-024-00240-y
- Park J, Park B, Ahn J, et al. Opto-ultrasound biosensor for wearable and mobile devices: realization with a transparent ultrasound transducer. Biomed Opt Express. 2022;13(9):4684-4692. doi: 10.1364/BOE.468969
- Park B, Han M, Park J, et al. A photoacoustic finder fully integrated with a solid-state dye laser and transparent ultrasound transducer. Photoacoustics. 2021;23:100290. doi: 10.1016/j.pacs.2021.100290
- Kim J, Lee J, Choi S, et al. 3d multiparametric photoacoustic computed tomography of primary and metastatic tumors in living mice. ACS Nano. 2024;18(28):18176-18190. doi: 10.1021/acsnano.3c12551
- Zhu X, Huang Q, DiSpirito A, et al. Real-time whole-brain imaging of hemodynamics and oxygenation at micro-vessel resolution with ultrafast wide-field photoacoustic microscopy. Light Sci Appl. 2022;11(1):138. doi: 10.1038/s41377-022-00836-2
- Park B, Bang C, Lee C, et al. 3D wide‐field multispectral photoacoustic imaging of human melanomas in vivo: a pilot study. J Eur Acad Dermatol Venereol. 2021;35(3):669-676. doi: 10.1111/jdv.16985
- Choi W, Park B, Choi S, Oh D, Kim J, Kim C. Recent advances in contrast-enhanced photoacoustic imaging: overcoming the physical and practical challenges. Chem Rev. 2023;123(11):7379-7419. doi: 10.1021/acs.chemrev.2c00627
- Park J, Park B, Kim TY, et al. Quadruple ultrasound, photoacoustic, optical coherence, and fluorescence fusion imaging with a transparent ultrasound transducer. Proc Natl Acad Sci USA. 2021;118(11):e1920879118. doi: 10.1073/pnas.1920879118
- Ma C, Li W, Li D, et al. Photoacoustic imaging of 3D-printed vascular networks. Biofabrication. 2022;14(2):025001. doi: 10.1088/1758-5090/ac49d5
- Monzel AS, Smits LM, Hemmer K, et al. Derivation of human midbrain-specific organoids from neuroepithelial stem cells. Stem Cell Reports. 2017;8(5):1144-1154. doi: 10.1016/j.stemcr.2017.03.010
- Breideband L, Wächtershäuser K, Hafa L, et al. Upgrading a consumer stereolithographic 3d printer to produce a physiologically relevant model with human liver cancer organoids. Adv Mater Technol. 2022;7(10):2200029. doi: 10.1002/admt.202200029
- Urciuolo A, Giobbe G, Dong Y, et al. Hydrogel-in-hydrogel live bioprinting for guidance and control of organoids and organotypic cultures. Nat Commun. 2023;14(1):3128. doi: 10.1038/s41467-023-37953-4
- Dong Q, Su X, Li X, et al. In vitro construction of lung cancer organoids by 3D bioprinting for drug evaluation. Coll Surf A Physicochem Eng Aspects. 2023;666:131288. doi: 10.1016/j.colsurfa.2023.131288
- Conrad C, Gray K, Stroka K, Rizvi I, Scarcelli G. Mechanical characterization of 3D ovarian cancer nodules using Brillouin confocal microscopy. Cell Mol Bioeng. 2019;12:215-226. doi: 10.1007/s12195-019-00570-7
- Coker Z, Troyanova M, Steelman Z, et al. Brillouin microscopy monitors rapid responses in subcellular compartments. Photonix. 2024;5(1):9. doi: 10.1186/s43074-024-00123-w
- Prevedel R, Diz-Muñoz A, Ruocco G, Antonacci G. Brillouin microscopy: an emerging tool for mechanobiology. Nat Methods. 2019;16(10):969-977. doi: 10.1038/s41592-019-0543-3
- Kabakova I, Zhang J, Xiang Y, et al. Brillouin microscopy. Nat Rev Methods Primers. 2024;4(1):8. doi: 10.1038/s43586-023-00286-z
- Caiaffa C, Tukeman G, Delgado C, et al. Dolutegravir induces FOLR1 expression during brain organoid development. Front Mol Neurosci. 2024;17:1394058. doi: 10.3389/fnmol.2024.1394058
- Bouvet P, Clément F, Papoz A, Dehoux T, Baritaux J. Multimode fiber-coupled VIPA spectrometer for high-throughput Brillouin imaging of biological samples. J Phys Photonics. 2024;6(2):025010. doi: 10.1088/2515-7647/ad378c
- Park J, Gao L. Advancements in fluorescence lifetime imaging microscopy Instrumentation: towards high speed and 3D. Curr Opin Solid State Mater Sci. 2024;30: 101147. doi: 10.1016/j.cossms.2024.101147
- Becker W. Fluorescence lifetime imaging–techniques and applications. J Microsc. 2012;247(2):119-136. doi: 10.1111/j.1365-2818.2012.03618.x
- Jakobs S, Subramaniam V, Schönle A, Jovin T, Hell S. EGFP and DsRed expressing cultures of Escherichia coli imaged by confocal, two-photon and fluorescence lifetime microscopy. FEBS Lett. 2000;479(3):131-135. doi: 10.1016/s0014-5793(00)01896-2
- Ma Y, Park J, Huang L, et al. Light-field tomographic fluorescence lifetime imaging microscopy. Proc Natl Acad Sci USA. 2024;121(40):e2402556121. doi: 10.1073/pnas.2402556121
- Barroso M, Monaghan M, Niesner R, Dmitriev R. Probing organoid metabolism using fluorescence lifetime imaging microscopy (FLIM): the next frontier of drug discovery and disease understanding. Adv Drug Deliv Rev. 2023: 115081. doi: 10.1016/j.addr.2023.115081
- Walsh A, Cook R, Sanders M, et al. Quantitative optical imaging of primary tumor organoid metabolism predicts drug response in breast cancer. Cancer Res. 2014;74(18):5184-5194. doi: 10.1158/0008-5472.CAN-14-0663
- Datta R, Heaster T, Sharick J, Gillette A, Skala M. Fluorescence lifetime imaging microscopy: fundamentals and advances in instrumentation, analysis, and applications. J Biomed Opt. 2020;25(7):071203-071203. doi: 10.1117/1.JBO.25.7.071203
- Sharma U, Chang E, Yun S. Long-wavelength optical coherence tomography at 1.7 μm for enhanced imaging depth. Opt Express. 2008;16(24):19712-19723. doi: 10.1364/oe.16.019712
- Gröhl J, Schellenberg M, Dreher K, Maier-Hein L. Deep learning for biomedical photoacoustic imaging: a review. Photoacoustics. 2021;22:100241. doi: 10.1016/j.pacs.2021.100241
- Fei K, Zhang J, Yuan J, Xiao P. Present application and perspectives of organoid imaging technology. Bioengineering. 2022;9(3):121. doi: 10.3390/bioengineering9030121
- Pettinato G, Goughlan M, Zhang X, et al. Spectroscopic label-free microscopy of changes in live cell chromatin and biochemical composition in transplantable organoids. Sci Adv. 2021;7(34):eabj2800. doi: 10.1126/sciadv.abj2800
- Errico C, Pierre J, Pezet S, et al. Ultrafast ultrasound localization microscopy for deep super-resolution vascular imaging. Nature. 2015;527:499-502. doi: 10.1038/nature16066
- Deben C, Hoz E, Compte M, et al. OrBITS: label-free and time-lapse monitoring of patient derived organoids for advanced drug screening. Cell Oncol. 2023;46(2):299-314. doi: 10.1007/s13402-022-00750-0
- Park E MS, Hwang D, et al. Unsupervised inter-domain transformation for virtually stained high-resolution mid-infrared photoacoustic microscopy using explainable deep learning. Nat Commun. 2024;(In press).