Improved osseointegration and segmental stability of 3D-printed porous tantalum cages with micro-scale structures for spinal fusion
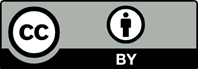
Spinal fusion surgery is an effective therapy for patients with disc herniation and degenerative disc disease. In this procedure, the intervertebral cage plays a key role in reconstructing stability and achieving fusion, though its clinical efficacy is limited by inadequate osseointegration. In this study, we developed a tantalum (Ta) cage, featuring micro-scale roughness and a porous microstructure, using advanced three-dimensional (3D) printing techniques. The aim of the study was to investigate its osteogenic potential in vitro and intervertebral fusion capability in vivo. Compared with conventional polyetheretherketone cages, in vitro biological experiments demonstrated that the 3D-printed porous Ta (3D-pTa) cages significantly enhanced osteoblast adhesion, proliferation, and differentiation. In vivo spinal fusion studies in a sheep model demonstrated significant increases in bone-implant contact and bone volume to total volume ratios (p < 0.05) with the 3D-pTa cages, indicating marked bone ingrowth and effective spinal fusion. Additionally, mechanical tests revealed that the 3D-pTa cages provided consistent stability and stiffness, significantly reducing the range of motion at various time points (p < 0.05). Our findings indicate that the 3D-pTa cage effectively facilitates bone fusion and possesses reliable biosafety, highlighting its potential for future clinical application in spinal surgery.

- Buser Z, Brodke DS, Youssef JA, et al. Synthetic bone graft versus autograft or allograft for spinal fusion: a systematic review. J Neurosurg Spine. 2016;25:509-516. doi: 10.3171/2016.1.Spine151005
- Hirvonen T, Siironen J, Marjamaa J, Niemela M, Koski- Palken A. Anterior cervical discectomy and fusion in young adults leads to favorable outcome in long-term follow-up. Spine J. 2020;20:1073-1084. doi: 10.1016/j.spinee.2020.03.016
- Gu X, Sun X, Sun Y, et al. Bioinspired modifications of PEEK implants for bone tissue engineering. Front Bioeng Biotechnol. 2020;8:631616. doi: 10.3389/fbioe.2020.631616
- Feng X, Yu H, Liu H, et al. Three-dimensionally-printed polyether-ether-ketone implant with a cross-linked structure and acid-etched microporous surface promotes integration with soft tissue. Int J Mol Sci. 2019;20:3811. doi: 10.3390/ijms20153811
- Buck E, Li H, Cerruti M. Surface modification strategies to improve the osseointegration of poly(etheretherketone) and its composites. Macromol Biosci. 2020;20:e1900271. doi: 10.1002/mabi.201900271
- Panayotov IV, Orti V, Cuisinier F, Yachouh J. Polyetheretherketone (PEEK) for medical applications. J Mater Sci Mater Med. 2016;27:1-11. doi: 10.1007/s10856-016-5731-4
- Kurtz SM, Devine JN. PEEK biomaterials in trauma, orthopedic, and spinal implants. Biomaterials. 2007;28:4845- 4869. doi: 10.1016/j.biomaterials.2007.07.013
- Toth JM, Wang M, Estes BT, Scifert JL, Seim HB, Turner AS. Polyetheretherketone as a biomaterial for spinal applications. Biomaterials. 2006;27:324-334. doi: 10.1016/j.biomaterials.2005.07.011
- Zhang W, Liu L, Zhou H, et al. Surface bisphosphonation of polyetheretherketone to manipulate immune response for advanced osseointegration. Mater Design. 2023; 232:112151. doi: 10.1016/j.matdes.2023.112151
- Al-Tamimi AA, Fernandes PRA, Peach C, Cooper G, Diver C, Bartolo PJ. Metallic bone fixation implants: a novel design approach for reducing the stress shielding phenomenon. Virtual Phys Prototy. 2017;12:141-151. doi: 10.1080/17452759.2017.1307769
- Pobloth AM, Checa S, Razi H, et al. Mechanobiologically optimized 3D titanium-mesh scaffolds enhance bone regeneration in critical segmental defects in sheep. Sci Transl Med. 2018;10:eaam8828. doi: 10.1126/scitranslmed.aam8828
- Turnbull G, Clarke J, Picard F, et al. 3D bioactive composite scaffolds for bone tissue engineering. Bioact Mater. 2018;3:278-314. doi: 10.1016/j.bioactmat.2017.10.001
- Levine BR, Sporer S, Poggie RA, Della Valle CJ, Jacobs JJ. Experimental and clinical performance of porous tantalum in orthopedic surgery. Biomaterials. 2006;27:4671-4681. doi: 10.1016/j.biomaterials.2006.04.041
- Sumner DR. Long-term implant fixation and stress-shielding in total hip replacement. J Biomech. 2015;48:797-800. doi: 10.1016/j.jbiomech.2014.12.021
- Tang Z, Xie YT, Yang F, et al. Porous tantalum coatings prepared by vacuum plasma spraying enhance BMSCs osteogenic differentiation and bone regeneration. PLoS One. 2013;8:e66263. doi: 10.1371/journal.pone.0066263
- Alipal J, Pu’ad NASM, Nayan NHM, et al. An updated review on surface functionalisation of titanium and its alloys for implants applications. Mater Today Proc. 2021; 42:270-282. doi: 10.1016/j.matpr.2021.01.499
- Han Q, Wang CY, Chen H, Zhao X, Wang JC. Porous Tantalum and titanium in orthopedics: a review. ACS Biomater Sci Eng. 2019;5:5798-5824. doi: 10.1021/acsbiomaterials.9b00493
- Sagomonyants KB, Hakim-Zargar M, Jhaveri A, Aronow MS, Gronowicz G. Porous tantalum stimulates the proliferation and osteogenesis of osteoblasts from elderly female patients. J Orthop Res. 2011;29:609-616. doi: 10.1002/jor.21251
- Huang G, Pan ST, Qiu JX. The clinical application of porous tantalum and its new development for bone tissue engineering. Materials. 2021;14:2647. doi: 10.3390/ma14102647
- Balla VK, Banerjee S, Bose S, Bandyopadhyay A. Direct laser processing of a tantalum coating on titanium for bone replacement structures. Acta Biomater. 2010; 6: 2329-2334. doi: 10.1016/j.actbio.2009.11.021
- Guo Y, Xie K, Jiang WB, et al. In vitro and in vivo study of 3D-printed porous tantalum scaffolds for repairing bone defects. ACS Biomater Sci Eng. 2019;5:1123-1133. doi: 10.1021/acsbiomaterials.8b01094
- Wang LN, Yuan B, Chen F, et al. Ability of a novel biomimetic titanium alloy cage in avoiding subsidence and promoting fusion: a goat spine model study. Mater Design. 2022;213:110361. doi: 10.1016/j.matdes.2021.110361
- Pei X, Wang LN, Wu LN, et al. In-situ synthesized hydroxyapatite whiskers on 3D printed titanium cages enhanced osteointegration in a goat spinal fusion model. Mater Design. 2023; 233: 112270. doi: 10.1016/j.matdes.2023.112270
- Laratta JL, Vivace BJ, Lopez-Pena M, et al. 3D-printed titanium cages without bone graft outperform PEEK cages with autograft in an animal model. Spine J. 2022; 22:1016- 1027. doi: 10.1016/j.spinee.2021.12.004
- Wang XY, Zhang DC, Peng HT, Yang JZ, Li Y, Xu JX. Optimize the pore size-pore distribution-pore geometry-porosity of 3D-printed porous tantalum to obtain optimal critical bone defect repair capability. Biomater Adv. 2023;154:213638. doi: 10.1016/j.bioadv.2023.213638
- Jiao JY, Hong QM, Zhang DC, et al. Influence of porosity on osteogenesis, bone growth and osteointegration in trabecular tantalum scaffolds fabricated by additive manufacturing. Front Bioeng Biotech. 2023;11:1117954. doi: 10.3389/fbioe.2023.1117954
- Wauthle R, van der Stok J, Amin Yavari S, et al. Additively manufactured porous tantalum implants. Acta Biomater. 2015;14:217-225. doi: 10.1016/j.actbio.2014.12.003
- Yang J, Gao H, Zhang D, et al. Static compressive behavior and material failure mechanism of trabecular tantalum scaffolds fabricated by laser powder bed fusion-based additive manufacturing. Int J Bioprint. 2022; 8: 438. doi: 10.18063/ijb.v8i1.438
- Jin J, Wang D, Qian H, et al. Precision pore structure optimization of additive manufacturing porous tantalum scaffolds for bone regeneration: a proof-of-concept study. Biomaterials. 2025;313:122756. doi: 10.1016/j.biomaterials.2024.122756
- Chen W, Yang J, Kong H, et al. Fatigue behaviour and biocompatibility of additively manufactured bioactive tantalum graded lattice structures for load-bearing orthopaedic applications. Mater Sci Eng C Mater Biol Appl. 2021;130:112461. doi: 10.1016/j.msec.2021.112461
- Yuan K, Zhang K, Yang YQ, et al. Evaluation of interbody fusion efficacy and biocompatibility of a polyetheretherketone/calcium silicate/porous tantalum cage in a goat model. J Orthop Transl. 2022;36:109-119. doi: 10.1016/j.jot.2022.06.006
- Zhang CX, Zhou ZW, Liu N, et al. Osteogenic differentiation of 3D-printed porous tantalum with nano-topographic modification for repairing craniofacial bone defects. Front Bioeng Biotech. 2023;11:1258030. doi: 10.3389/fbioe.2023.1258030
- Fan HQ, Deng S, Tang WT, et al. Highly porous 3D printed tantalum scaffolds have better biomechanical and microstructural properties than titanium scaffolds. Biomed Res Int. 2021;2021:2899043. doi: 10.1155/2021/2899043
- Cheers GM, Weimer LP, Neuerburg C, et al. Advances in implants and bone graft types for lumbar spinal fusion surgery. Biomater Sci. 2024;12:4875-4902. doi: 10.1039/d4bm00848k
- de Silva L, Bernal PN, Rosenberg A, Malda J, Levato R, Gawlitta D. Biofabricating the vascular tree in engineered bone tissue. Acta Biomater. 2023;156:250-268. doi: 10.1016/j.actbio.2022.08.051
- Loenen ACY, Peters MJM, Bevers RTJ, et al. Early bone ingrowth and segmental stability of a trussed titanium cage versus a polyether ether ketone cage in an ovine lumbar interbody fusion model. Spine J. 2022;22:174-182. doi: 10.1016/j.spinee.2021.07.011
- Li MM, Yin SH, Lin MZ, et al. Current status and prospects of metal-organic frameworks for bone therapy and bone repair. J Mater Chem B. 2022;10:5105-528. doi: 10.1039/d2tb00742h
- Huang G, Pan ST, Qiu JX. The osteogenic effects of porous Tantalum and Titanium alloy scaffolds with different unit cell structure. Colloid Surface B. 2022;210:112229. doi: 10.1016/j.colsurfb.2021.112229
- Babuska V, Kasi PB, Chocholata P, et al. Nanomaterials in bone regeneration. Appl Sci Basel. 2022;12:6793. doi: 10.3390/app12136793
- Barberi J, Spriano S. Titanium and protein adsorption: an overview of mechanisms and effects of surface features. Materials (Basel). 2021;14:1590. doi: 10.3390/ma14071590
- Ji S, Guvendiren M. 3D printed wavy scaffolds enhance mesenchymal stem cell osteogenesis. Micromachines (Basel). 2019;11:31. doi: 10.3390/mi11010031
- Wang X, Liu W, Yu X, et al. Advances in surface modification of tantalum and porous tantalum for rapid osseointegration: a thematic review. Front Bioeng Biotechnol. 2022; 10:983695. doi: 10.3389/fbioe.2022.983695
- McGilvray KC, Easley J, Seim HB, et al. Bony ingrowth potential of 3D-printed porous titanium alloy: a direct comparison of interbody cage materials in an in vivo ovine lumbar fusion model. Spine J. 2018;18:1250-1260. doi: 10.1016/j.spinee.2018.02.018
- Xu CC, Wu FH, Yang J, et al. 3D printed long-term structurally stable bioceramic dome scaffolds with controllable biodegradation favorable for guided bone regeneration. Chem Eng J. 2022; 450:138003. doi: 10.1016/j.cej.2022.138003
- Wei XH, Zhou WH, Tang Z, et al. Magnesium surface-activated 3D printed porous PEEK scaffolds for osseointegration by promoting angiogenesis and osteogenesis. Bioact Mater. 2023;20:16-28. doi: 10.1016/j.bioactmat.2022.05.011
- Qian H, Lei T, Hua L, et al. Fabrication, bacteriostasis and osteointegration properties researches of the additively-manufactured porous tantalum scaffolds loading vancomycin. Bioact Mater. 2023;24:450-462. doi: 10.1016/j.bioactmat.2022.12.013
- Liguori A, Gino ME, Panzavolta S, et al. Tantalum nanoparticles enhance the osteoinductivity of multiscale composites based on poly(lactide-co-glycolide) electrospun fibers embedded in a gelatin hydrogel. Mater Today Chem. 2022;24:100804. doi: 10.1016/j.mtchem.2022.100804
- Jia CQ, Zhang Z, Cao SQ, et al. A biomimetic gradient porous cage with a micro-structure for enhancing mechanical properties and accelerating osseointegration in spinal fusion. Bioact Mater. 2023;23:234-246. doi: 10.1016/j.bioactmat.2022.11.003
- Feng XB, Ma L, Liang H, et al. Osteointegration of 3D-printed fully porous polyetheretherketone scaffolds with different pore sizes. ACS Omega. 2020;5:26655-26666. doi: 10.1021/acsomega.0c03489
- Shen YW, Yang Y, Liu H, et al. Preliminary results in anterior cervical discectomy and fusion with the uncovertebral joint fusion cage in a goat model. BMC Musculoskel Dis. 2021;22:1-10. doi: 10.1186/s12891-021-04412-4
- Sheng SR, Wang XY, Xu HZ, Zhu GQ, Zhou YF. Anatomy of large animal spines and its comparison to the human spine: a systematic review. Eur Spine J. 2010;19:46-56. doi: 10.1007/s00586-009-1192-5
- Kandziora F, Pflugmacher R, Scholz M, et al. Comparison between sheep and human cervical spines: an anatomic, radiographic, bone mineral density, and biomechanical study. Spine (Phila Pa 1976). 2001;26:1028-1037. doi: 10.1097/00007632-200105010-00008
- Godlewski B, Bebenek A, Dominiak M, Karpinski G, Cieslik P, Pawelczyk T. Subsidence following cervical discectomy and implant-to-bone ratio. BMC Musculoskelet Disord. 2022;23:750. doi: 10.1186/s12891-022-05698-8
- Mathieu L, Marie-Solenne F, Cécile B, et al. Biocompatibility of four common orthopedic biomaterials following neuroelectromyostimulation: an study. J Biomed Mater Res B. 2018;106:1156-1164. doi: 10.1002/jbm.b.33927
- Bernard M, Jubeli E, Pungente MD, Yagoubi N. Biocompatibility of polymer-based biomaterials and medical devices - regulations, screening and risk-management. Biomater Sci Uk 2018;6:2025-2053. doi: 10.1039/c8bm00518d