Parametric computational modeling of melt electrowritten scaffolds: Predicting the cellular micromechanical environment for gradient tissue engineering in rotator cuff repair
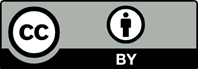
Surgical interventions of the rotator cuff (RC) tendons are failing at unacceptably high rates. This is primarily due to clinical strategies failing to regenerate the biomechanical junction between bone and tendon (enthesis). Tissue engineering approaches for RC repair involve attempts at increasing the acute strength of the repair and directing the healing cascade to regenerate the enthesis. Advances in bioprinting, specifically melt electrowriting (MEW), allow precise fabrication of microarchitectures. Our group has utilized the functionality MEW offers to create a novel, single-material, gradient scaffold that approaches the mechanical gradients present in the RC. To characterize this novel geometry, high-throughput, parametric finite element analysis (FEA) models were generated: (1) to determine how tunable print parameters such as the radius of curvature of fibers, the fiber diameter, and the fiber spacing alter the gradient present in the scaffold’s architecture; and (2) to predict, at the cellular level, what strain the scaffold generates regionally to ``instruct’’ the local cell milieu to produce healthy tissues (e.g., bone and tendon). FEA models predicted that mechanical gradients in the scaffold approached gradient levels seen in the RC (≈2 orders of magnitude), in which global strain gradients were driven by the radius of curvature of fibers. Additionally, our novel architecture significantly affected regional mechanics, which could be optimized for tenocyte and osteoblastic bioactivity. The data presented demonstrate a novel, tunable architecture capable of approaching biologically relevant gradients observed in the RC with potential to address the clinical problems associated with tendon–bone repairs in other regions of the body using only a single material.

- Colvin AC, Egorova N, Harrison AK, Moskowitz A, Flatow EL. National trends in rotator cuff repair. J Bone Joint Surg Am. 2012;94(3):227-233. doi: 10.2106/JBJS.J.00739
- Galatz LM, Ball CM, Teefey SA, Middleton WD, Yamaguchi K. The outcome and repair integrity of completely arthroscopically repaired large and massive rotator cuff tears. JBJS. 2004;86(2); 219-224. doi: 10.2106/00004623-200402000-00002
- Zhang X, Wang D, Wang Z, et al. Clinical perspectives for repairing rotator cuff injuries with multi-tissue regenerative approaches. J Orthop Translat. 2022;36:91-108. doi: 10.1016/j.jot.2022.06.004
- Zumstein MA, Lädermann A, Raniga S, Schär MO. The biology of rotator cuff healing. Orthop Traumatol Surg Res. 2017;103(1S):S1-S10. doi: 10.1016/j.otsr.2016.11.003
- Iannotti JP, Codsi MJ, Kwon YW, Derwin K, Ciccone J, Brems JJ. Porcine small intestine submucosa augmentation of surgical repair of chronic two-tendon rotator cuff tears: a randomized, controlled trial. JBJS. 2006;88(6):1238-1244. doi: 10.2106/jbjs.E.00524
- Malcarney HL, Bonar F, Murrell GAC. Early inflammatory reaction after rotator cuff repair with a porcine small intestine submucosal implant:a report of 4 cases. Am J Sports Med. 2005;33(6):907-911. doi: 10.1177/0363546504271500
- Mobarak MH, Islam MA, Hossain N, et al. Recent advances of additive manufacturing in implant fabrication – a review. Appl Surf Sci Adv. 2023;18:100462. doi: 10.1016/j.apsadv.2023.100462
- Yang Y, Wang G, Liang H, et al. Additive manufacturing of bone scaffolds. IJB. 2018;5(1):148. doi: 10.18063/ijb.v5i1.148
- Wu Y, Liu J, Kang L, et al. An overview of 3D printed metal implants in orthopedic applications: present and future perspectives. Heliyon. 2023;9(7):e17718. doi: 10.1016/j.heliyon.2023.e17718
- Kotrych D, Angelini A, Bohatyrewicz A, Ruggieri P. 3D printing for patient-specific implants in musculoskeletal oncology. EFORT Open Rev. 2023;8(5):331-339. doi: 10.1530/EOR-23-0066
- Chen A, Su J, Li Y, et al. 3D/4D printed bio-piezoelectric smart scaffolds for next-generation bone tissue engineering. Int J Extrem Manuf. 2023;5(3):032007. doi: 10.1088/2631-7990/acd88f
- Federici AS, Tornifoglio B, Lally C, Garcia O, Kelly DJ, Hoey DA. Melt electrowritten scaffold architectures to mimic tissue mechanics and guide neo-tissue orientation. J Mech Behav Biomed Mater. 2024;150:106292. doi: 10.1016/j.jmbbm.2023.106292
- Kaspar D, Seidl W, Neidlinger-Wilke C, Claes L. In vitro effects of dynamic strain on the proliferative and metabolic activity of human osteoblasts. J Musculoskelet Neuronal Interact. 2000;1(2):161-164
- Zhu J, Zhang X, Wang C, Peng X, Zhang X. Different magnitudes of tensile strain induce human osteoblasts differentiation associated with the activation of ERK1/2 phosphorylation. Int J Mol Sci. 2008;9(12):2322-2332. doi: 10.3390/ijms9122322
- Yan Y-x, Gong Y-w, Guo Y, et al. Mechanical strain regulates osteoblast proliferation through integrin-mediated ERK activation. PLOS ONE. 2012;7(4):e35709. doi: 10.1371/journal.pone.0035709
- Guo Y, Zhang C-q, Zeng Q-c, et al. Mechanical strain promotes osteoblast ECM formation and improves its osteoinductive potential. BioMed Eng OnLine. 2012;11(1):80. doi: 10.1186/1475-925X-11-80
- Burger EH, Klein-Nulend J. Mechanotransduction in bone—role of the lacunocanalicular network. FASEB J. 1999;13(9001):S101-S112. doi: 10.1096/fasebj.13.9001.s101
- Claes LE, Heigele CA. Magnitudes of local stress and strain along bony surfaces predict the course and type of fracture healing. J Biomechan. 1999;32(3):255-266. doi: 10.1016/S0021-9290(98)00153-5
- Schoenenberger AD, Tempfer H, Lehner C, et al. Macromechanics and polycaprolactone fiber organization drive macrophage polarization and regulate inflammatory activation of tendon in vitro and in vivo. Biomaterials. 2020;249:120034. doi: 10.1016/j.biomaterials.2020.120034
- Wu S, Peng H, Li X, Streubel PN, Liu Y, Duan B. Effect of scaffold morphology and cell co-culture on tenogenic differentiation of HADMSC on centrifugal melt electrospun poly (L‑lactic acid) fibrous meshes. Biofabrication. 2017;9(4):044106-044106. doi: 10.1088/1758-5090/aa8fb8
- El Khatib MA-O, Mauro AA-O, Di Mattia MA-O, et al. Electrospun PLGA fiber diameter and alignment of tendon biomimetic fleece potentiate tenogenic differentiation and immunomodulatory function of amniotic epithelial stem cells. Cells. 2020;9(5):1207. doi: 10.3390/cells9051207
- Yang G, Im H-J, Wang JHC. Repetitive mechanical stretching modulates IL-1β induced COX-2, MMP-1 expression, and PGE2 production in human patellar tendon fibroblasts. Gene. 2005;363:166-172. doi: 10.1016/j.gene.2005.08.006
- Wang T, Lin Z, Day RE, et al. Programmable mechanical stimulation influences tendon homeostasis in a bioreactor system. Biotechnol Bioeng. 2013;110(5):1495-1507. doi: 10.1002/bit.24809
- Yang G, Crawford RC, Wang JHC. Proliferation and collagen production of human patellar tendon fibroblasts in response to cyclic uniaxial stretching in serum-free conditions. J Biomech. 2004;37(10):1543-1550. doi: 10.1016/j.jbiomech.2004.01.005
- Chen Y, Hao M, Bousso I, Thomopoulos S, Xia Y. Reliable fabrication of mineral-graded scaffolds by spin-coating and laser machining for use in tendon-to-bone insertion repair. Adv Healthc Mater. 2024;13:e2402531. doi: 10.1002/adhm.202402531
- Spalazzi JP, Dagher E, Doty SB, Guo XE, Rodeo SA, Lu HH. In vivo evaluation of a multiphased scaffold designed for orthopaedic interface tissue engineering and soft tissue-to-bone integration. J Biomed Mater Res A. 2008;86(1):1-12. doi: 10.1002/jbm.a.32073
- Chen C, Shi Q, Li M, et al. Engineering an enthesis-like graft for rotator cuff repair: an approach to fabricate highly biomimetic scaffold capable of zone-specifically releasing stem cell differentiation inducers. Bioact Mater. 2022;16:451-471. doi: 10.1016/j.bioactmat.2021.12.021
- Xie J, Li X, Lipner J, et al. “Aligned-to-random” nanofiber scaffolds for mimicking the structure of the tendon-to-bone insertion site. Nanoscale. 2010;2(6):923-926. doi: 10.1039/c0nr00192a
- Tindell RK, Busselle LP, Holloway JL. Magnetic fields enable precise spatial control over electrospun fiber alignment for fabricating complex gradient materials. J Biomed Mater Res A. 2023;111(6):778-789. doi: 10.1002/jbm.a.37492
- Xiong J, Wang H, Lan X, et al. Fabrication of bioinspired grid-crimp micropatterns by melt electrospinning writing for bone–ligament interface study. Biofabrication. 2022;14(2):025008. doi: 10.1088/1758-5090/ac4ac8
- Jun I, Han H-S, Edwards JR, Jeon H. Electrospun fibrous scaffolds for tissue engineering: viewpoints on architecture and fabrication. Int J Mol Sci. 2018;19(3):745. doi: 10.3390/ijms19030745
- Abbasi N, Abdal-hay A, Hamlet S, Graham E, Ivanovski S. Effects of gradient and offset architectures on the mechanical and biological properties of 3-d melt electrowritten (MEW) scaffolds. ACS Biomater Sci Eng. 2019;5(7):3448-3461. doi: 10.1021/acsbiomaterials.8b01456
- Golafshan N, Castilho M, Daghrery A, et al. Composite graded melt electrowritten scaffolds for regeneration of the periodontal ligament-to-bone interface. ACS Appl Mater Interfaces. 2023;15(10):12735-12749. doi: 10.1021/acsami.2c21256
- Saiz PG, Reizabal A, Vilas-Vilela JL, Dalton PD, Lanceros- Mendez S. Materials and strategies to enhance melt electrowriting potential. Adv Mater. 2024;36(24):2312084. doi: 10.1002/adma.202312084
- Page MI, Linde PE, Puttlitz CM. High throughput computational evaluation of how scaffold architecture, material selection, and loading modality influence the cellular micromechanical environment in tissue engineering strategies. JOR SPINE. 2021;4(3):e1152. doi: 10.1002/jsp2.1152
- Page M, Puttlitz C. Biaxial mechanics of 3D fiber deposited ply-laminate scaffolds for soft tissue engineering part II: finite element analyses. J Mech Behav Biomed Mater. 2019;100:103395. doi: 10.1016/j.jmbbm.2019.103395
- Samavedi S, Olsen Horton C, Guelcher SA, Goldstein AS, Whittington AR. Fabrication of a model continuously graded co-electrospun mesh for regeneration of the ligament–bone interface. Acta Biomater. 2011;7(12):4131-4138. doi: 10.1016/j.actbio.2011.07.008
- Dwivedi R, Kumar S, Pandey R, Mahajan A, Nandana D, Katti DS, Mehrotra D. Polycaprolactone as biomaterial for bone scaffolds: Review of literature. J Oral Biol Craniofac Res. 2020;10(1):381-388. doi: 10.1016/j.jobcr.2019.10.003
- Polak-Krasna K, Mazgajczyk E, Heikkila P, Georgiadis A. Parametric finite element model and mechanical characterisation of electrospun materials for biomedical applications. Materials (Basel). 2021;14(2):278. doi: 10.3390/ma14020278
- Eshraghi S, Das S. Mechanical and microstructural properties of polycaprolactone scaffolds with one-dimensional, two-dimensional, and three-dimensional orthogonally oriented porous architectures produced by selective laser sintering. Acta Biomater. 2010;6(7):2467-2476. doi: 10.1016/j.actbio.2010.02.002
- Wolynski JG, Ilić MM, Labus KM, Notaroš BM, Puttlitz CM, McGilvray KC. Direct electromagnetic coupling to determine diagnostic bone fracture stiffness. Ann Transl Med. 2022;10(9):510. doi: 10.21037/atm-21-5315
- Tourlomousis F, Ding H, Kalyon DM, Chang RC. Melt electrospinning writing process guided by a “Printability Number”. J Manuf Sci Eng. 2017;139(8):081004. doi: 10.1115/1.4036348
- Eichholz KF, Hoey DA. Mediating human stem cell behaviour via defined fibrous architectures by melt electrospinning writing. Acta Biomater. 2018;75:140-151. doi: 10.1016/j.actbio.2018.05.048
- Jenkins TL, Little D. Synthetic scaffolds for musculoskeletal tissue engineering: cellular responses to fiber parameters. NPJ Regen Med. 2019;4:15. doi: 10.1038/s41536-019-0076-5
- Erisken C, Zhang X, Moffat KL, Levine WN, Lu HH. Scaffold fiber diameter regulates human tendon fibroblast growth and differentiation. Tissue Eng Part A. 2013;19(3-4):519-528. doi: 10.1089/ten.tea.2012.0072
- Kim J, Bakirci E, O’Neill KL, Hrynevich A, Dalton PD. Fiber bridging during melt electrowriting of poly(ε-Caprolactone) and the influence of fiber diameter and wall height. Macromol Mater Eng. 2021;306(3):2000685. doi: 10.1002/mame.202000685
- Morgan EF, Unnikrisnan GU, Hussein AI. Bone mechanical properties in healthy and diseased states. Annu Rev Biomed Eng. 2018;20:119-143. doi: 10.1146/annurev-bioeng-062117-121139
- Fratzl P. Collagen: Structure and Mechanics; New York, NY: Springer; 2008:1-506.
- Itoi E, Berglund LJ, Grabowski JJ, et al. Tensile properties of the supraspinatus tendon. J Orthop Res. 1995;13(4): 578-584. doi: 10.1002/jor.1100130413
- Kolel A, Ergaz B, Goren S, Tchaicheeyan O, Lesman A. Strain gradient programming in 3D fibrous hydrogels to direct graded cell alignment. Small Methods. 2023;7(1):2201070. doi: 10.1002/smtd.202201070
- Vader D, Kabla A, Weitz D, Mahadevan L. Strain-induced alignment in collagen gels. PLoS One. 2009;4(6):e5902. doi: 10.1371/journal.pone.0005902
- Cordin M, Bechtold T, Pham T. Effect of fibre orientation on the mechanical properties of polypropylene–lyocell composites. Cellulose. 2018;25(12):7197-7210. doi: 10.1007/s10570-018-2079-6
- Engler AJ, Sen S, Sweeney HL, Discher DE. Matrix elasticity directs stem cell lineage specification. Cell. 2006;126(4):677-689. doi: 10.1016/j.cell.2006.06.044
- Singh D, Rana A, Jhajhria SK, Garg B, Pandey PM, Kalyanasundaram D. Experimental assessment of biomechanical properties in human male elbow bone subjected to bending and compression loads. J Appl Biomater Funct Mater. 2019;17(2):2280800018793816. doi: 10.1177/2280800018793816
- Chae S, Yong U, Park W, et al. 3D cell-printing of gradient multi-tissue interfaces for rotator cuff regeneration. Bioact Mater. 2023;19:611-625. doi: 10.1016/j.bioactmat.2022.05.004
- Credille KT, Wang ZRC, Horner NS, et al. Biphasic interpositional allograft for rotator cuff repair augmentation is safe in an ovine model. Arthroscopy. 2023;39(9):1983-1997. doi: 10.1016/j.arthro.2023.03.018
- Korpershoek JV, Ruijter M, Terhaard BF, et al. Potential of melt electrowritten scaffolds seeded with meniscus cells and mesenchymal stromal cells. Int J Mol Sci. 2021;22(20):11200. doi: 10.3390/ijms222011200
- Xu T, Gu J, Meng J, Du L, Kumar A, Xu H. Melt electrowriting reinforced composite membrane for controlled drug release. J Mech Behav Biomed Mater. 2022;132:105277. doi: 10.1016/j.jmbbm.2022.105277
- Nieuwoudt M, Woods I, Eichholz KF, et al. Functionalization of electrospun polycaprolactone scaffolds with matrix-binding osteocyte-derived extracellular vesicles promotes osteoblastic differentiation and mineralization. Ann Biomed Eng. 2021;49(12):3621-3635. doi: 10.1007/s10439-021-02872-2
- Hrynevich A, Elçi BŞ, Haigh JN, et al. Dimension-based design of melt electrowritten scaffolds. Small. 2018;14(22): 1800232. doi: 10.1002/smll.201800232
- Loewner S, Heene S, Baroth T, et al. Recent advances in melt electro writing for tissue engineering for 3D printing of microporous scaffolds for tissue engineering. Front Bioeng Biotechnol. 2022;10:896719. doi: 10.3389/fbioe.2022.896719
- Mifsud T, Chatzistergos P, Maganaris C, et al. Supersonic shear wave elastography of human tendons is associated with in vivo tendon stiffness over small strains. J Biomech. 2023;152:111558. doi: 10.1016/j.jbiomech.2023.111558
- Reizabal A, Kangur T, Saiz PG, et al. MEWron: an open-source melt electrowriting platform. Additive Manuf. 2023;71:103604. doi: 10.1016/j.addma.2023.103604
- Apostolakos J, Durant TJ, Dwyer CR, et al. The enthesis: a review of the tendon-to-bone insertion. Muscles Ligaments Tendons J. 2014;4(3):333-342.
- Abdal-hay A, Kocak-Oztug NA, Sheikh FA, et al. Fabrication of 3D bioactive melt electrowriting composite scaffold with high osteogenic potential. Colloids Surf B: Biointerfaces. 2025;245:114270. doi: 10.1016/j.colsurfb.2024.114270
- Ielo I, Calabrese G, De Luca G, Conoci S. Recent advances in hydroxyapatite-based biocomposites for bone tissue regeneration in orthopedics. Int J Mol Sci. 2022;23(17): 9721. doi: 10.3390/ijms23179721
- Sgroi TA, Cilenti M. Rotator cuff repair: post-operative rehabilitation concepts. Curr Rev Musculoskelet Med. 2018;11(1):86-91. doi: 10.1007/s12178-018-9462-7
- Bas O, D’Angella D, Baldwin JG, et al. An integrated design, material, and fabrication platform for engineering biomechanically and biologically functional soft tissues. ACS Appl Mater Interfaces. 2017;9(35):29430-29437. doi: 10.1021/acsami.7b08617
- Richardson WJ, Kegerreis B, Thomopoulos S, Holmes JW. Potential strain-dependent mechanisms defining matrix alignment in healing tendons. Biomech Model Mechanobiol. 2018;17(6):1569-1580. doi: 10.1007/s10237-018-1044-5
- Chen K, Hu X, Blemker SS, Holmes JW. Multiscale computational model of Achilles tendon wound healing: Untangling the effects of repair and loading. PLoS Comput Biol. 2018;14(12):e1006652. doi: 10.1371/journal.pcbi.1006652
- Wittkowske C, Reilly GC, Lacroix D, Perrault CM. In vitro bone cell models: impact of fluid shear stress on bone formation. Front Bioeng Biotechnol. 2016;4:87. doi: 10.3389/fbioe.2016.00087