Nanomaterial-modified bioinks for DLP-based bioprinting of bone constructs: Impact on mechanical properties and mesenchymal stem cell function
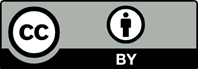
3D printing technologies offer tremendous potential to produce patient-specific implants and treat critical-sized bone defects, which vary in size, shape, and clinical requirements. Despite advancements in 3D printing of biomaterial-based bone constructs, they often lack biologically active material. For larger-sized bone implants, early biologization and vascularization are essential. In this context, bioprinting technologies enable the integration of vital cells or active growth factors into 3D-printed constructs, while the integration of nanomaterials enables material-mediated functionalization of the bioink. To date, such bioink modifications with nanomaterials have rarely been reported for digital light processing (DLP) bioprinting technology. Furthermore, there is a notable lack of direct comparative studies on the impact of nanomaterials on cellular processes. In this study, we assessed and compared graphene oxide (GO)- and calcium phosphate (CaP)-modified bioinks for DLP bioprinting of methacrylated gelatin (GelMa)-based bone constructs. After printing, the impact of bioinks on cell distribution, viability, cell proliferation, and differentiation, as well as the mechanical and structural properties of constructs, was evaluated. In comparison to commercial bioinks, cell viability was higher in the established GelMa bioinks. Morphological data and DNA quantification indicate the highest cell vitality and proliferation over time in basic GelMa bioink. CaP-modified GelMa bioink displayed the highest differentiation of human mesenchymal stem cells (hMSCs), in terms of osteogenic gene expression and calcium deposition. Conversely, GO increased the Young’s modulus of the material, affecting cell morphology. Overall, the direct comparison of nanomaterials suggests diverse effects in functionalizing DLP-printed bone constructs containing living osteogenic cells.

- Maruyama M, Rhee C, Utsunomiya T, et al. Modulation of the inflammatory response and bone healingr Review. Front Endocrinol. 2020;11:386. doi: 10.3389/fendo.2020.00386
- Ko HF, Sfeir C, Kumta PN. Novel synthesis strategies for natural polymer and composite biomaterials as potential scaffolds for tissue engineering. Philos Trans A Math Phys Eng Sci. 2010;368(1917):1981-1997. doi: 10.1098/rsta.2010.0009
- LeGeros RZ. Properties of osteoconductive biomaterials: calcium phosphates. Clin Orthop Relat Res. 2002;395:81-98. doi: 10.1097/00003086-200202000-00009
- Dimitriou R, Mataliotakis GI, Angoules AG, Kanakaris NK, Giannoudis PV. Complications following autologous bone graft harvesting from the iliac crest and using the RIA: a systematic review. Injury. 2011;42:S3-S15. doi: 10.1016/j.injury.2011.06.015
- Nauth A, Schemitsch E, Norris B, Nollin Z, Watson JT. Critical-size bone defects: is there a consensus for diagnosis and treatment? J Orthop Trauma. 2018;32(Suppl 1):S7-S11. doi: 10.1097/bot.0000000000001115
- Aktuglu K, Erol K, Vahabi A. Ilizarov bone transport and treatment of critical-sized tibial bone defects: a narrative review. J Orthop Traumatol. 2019;20(1):22. doi: 10.1186/s10195-019-0527-1
- Zhu W, Ma X, Gou M, Mei D, Zhang K, Chen S. 3D printing of functional biomaterials for tissue engineering. Curr Opin Biotechnol. 2016;40:103-112. doi: 10.1016/j.copbio.2016.03.014
- Haglin JM, Eltorai AE, Gil JA, Marcaccio SE, Botero- Hincapie J, Daniels AH. Patient-specific orthopaedic implants. Orthop Surg. 2016;8(4):417-424. doi: 10.1111/os.12282
- Mobbs RJ, Parr WCH, Huang C, Amin T. Rapid personalised virtual planning and on-demand surgery for acute spinal trauma using 3D-printing, biomodelling and patient-specific implant manufacture. J Pers Med. 2022; 12(6):997. doi: 10.3390/jpm12060997
- Ozbolat IT, Hospodiuk M. Current advances and future perspectives in extrusion-based bioprinting. Biomaterials. 2016;76:321-343. doi: 10.1016/j.biomaterials.2015.10.076
- Liang R, Gu Y, Wu Y, Bunpetch V, Zhang S. Lithography-based 3D bioprinting and bioinks for bone repair and regeneration. ACS Biomater Sci Eng. 2021;7(3): 806-816. doi: 10.1021/acsbiomaterials.9b01818
- Kim SH, Kim DY, Lim TH, Park CH. Silk fibroin bioinks for digital light processing (DLP) 3D bioprinting. Adv Exp Med Biol. 2020;1249:53-66. doi: 10.1007/978-981-15-3258-0_4
- Shen Y, Tang H, Huang X, et al. DLP printing photocurable chitosan to build bio-constructs for tissue engineering. Carbohydr Polym. 2020;235:115970. doi: 10.1016/j.carbpol.2020.115970
- Sheng L, Li M, Zheng S, Qi J. Adjusting the accuracy of PEGDA-GelMA vascular network by dark pigments via digital light processing printing. J. Biomater Appl. 2022;36(7):1173-1187. doi: 10.1177/08853282211053081
- Song P, Gui X, Wu L, et al. DLP fabrication of multiple hierarchical biomimetic GelMA/SilMA/HAp scaffolds for enhancing bone regeneration. Biomacromolecules. 2024;25(3):1871-1886. doi: 10.1021/acs.biomac.3c01318
- Tao J, Zhu S, Liao X, et al. DLP-based bioprinting of void-forming hydrogels for enhanced stem-cell-mediated bone regeneration. Mater Today Bio. 2022;17:100487. doi: 10.1016/j.mtbio.2022.100487
- Park SH, Park DS, Shin JW, et al. Scaffolds for bone tissue engineering fabricated from two different materials by the rapid prototyping technique: PCL versus PLGA. J Mater Sci Mater Med. 2012;23(11):2671-2678. doi: 10.1007/s10856-012-4738-8
- Tibbitt MW, Rodell CB, Burdick JA, Anseth KS. Progress in material design for biomedical applications. Proc Natl Acad Sci. 2015;112(47):14444-14451. doi: 10.1073/pnas.1516247112
- Nguyen DG, Funk J, Robbins JB, et al. Bioprinted 3D primary liver tissues allow assessment of organ-level response to clinical drug induced toxicity in vitro. Plos One. 2016;11(7):e0158674. doi: 10.1371/journal.pone.0158674
- Lee KY, Mooney DJ. Hydrogels for tissue engineering. Chem Rev. 2001;101(7):1869-1880. doi: 10.1021/cr000108x
- Levato R, Visser J, Planell JA, Engel E, Malda J, Mateos- Timoneda MA. Biofabrication of tissue constructs by 3D bioprinting of cell-laden microcarriers. Biofabrication. 2014;6(3):035020. doi: 10.1088/1758-5082/6/3/035020
- Leijten J, Rouwkema J, Zhang YS, Nasajpour A, Dokmeci MR, Khademhosseini A. Advancing tissue engineering: a tale of nano-, micro-, and macroscale integration. Small. 2016;12(16):2130-2145. doi: 10.1002/smll.201501798
- Wüst S, Müller R, Hofmann S. Controlled positioning of cells in biomaterials—approaches towards 3D tissue printing. J Funct Biomater. 2011;2(3):119-154. doi: 10.3390/jfb2030119
- Murphy SV, Atala A. 3D bioprinting of tissues and organs. Nat Biotechnol. 2014;32(8):773-785. doi: 10.1038/nbt.2958
- Liu Y, Chan-Park MB. A biomimetic hydrogel based on methacrylated dextran-graft-lysine and gelatin for 3D smooth muscle cell culture. Biomaterials. 2010;31(6):1158-1170. doi: 10.1016/j.biomaterials.2009.10.040
- Jeong HJ, Nam H, Jang J, Lee SJ. 3D Bioprinting strategies for the regeneration of functional tubular tissues and organs. Bioengineering (Basel). 2020;7(2):32. doi: 10.3390/bioengineering7020032
- Xu T, Zhao W, Zhu J-M, Albanna MZ, Yoo JJ, Atala A. Complex heterogeneous tissue constructs containing multiple cell types prepared by inkjet printing technology. Biomaterials. 2013;34(1):130-139. doi: 10.1016/j.biomaterials.2012.09.035
- Li J, Mooney DJ. Designing hydrogels for controlled drug delivery. Nat Rev Mater. 2016;1(12):16071. doi: 10.1038/natrevmats.2016.71
- Gao J, Li M, Cheng J, et al. 3D-printed GelMA/PEGDA/ F127DA scaffolds for bone regeneration. J Funct Biomater. 2023;14(2):96. doi: 10.3390/jfb14020096
- Gao J, Wang H, Li M, et al. DLP-printed GelMA-PMAA scaffold for bone regeneration through endochondral ossification. Int J Bioprint. 2023;9(5):754. doi: 10.18063/ijb.754
- Shi H, Li Y, Xu K, Yin J. Advantages of photo-curable collagen-based cell-laden bioinks compared to methacrylated gelatin (GelMA) in digital light processing (DLP) and extrusion bioprinting. Mater Today Bio. 2023;23:100799. doi: 10.1016/j.mtbio.2023.100799
- Kurian AG, Singh RK, Patel KD, Lee JH, Kim HW. Multifunctional GelMA platforms with nanomaterials for advanced tissue therapeutics. Bioact Mater. 2022;8: 267-295. doi: 10.1016/j.bioactmat.2021.06.027
- Li J, Moeinzadeh S, Kim C, et al. Development and systematic characterization of GelMA/alginate/PEGDMA/xanthan gum hydrogel bioink system for extrusion bioprinting. Biomaterials. 2023;293:121969. doi: 10.1016/j.biomaterials.2022.121969
- Pérez-Cortez JE, Sánchez-Rodríguez VH, Gallegos- Martínez S, et al. Low-cost light-based GelMA 3D bioprinting via retrofitting: manufacturability test and cell culture assessment. Micromachines (Basel). 2022; 14(1):55. doi: 10.3390/mi14010055
- Sun X, Ma Z, Zhao X, et al. Three-dimensional bioprinting of multicell-laden scaffolds containing bone morphogenic protein-4 for promoting M2 macrophage polarization and accelerating bone defect repair in diabetes mellitus. Bioact Mater. 2021;6(3):757-769. doi: 10.1016/j.bioactmat.2020.08.030
- Yi S, Liu Q, Luo Z, et al. Micropore-forming gelatin methacryloyl (GelMA) bioink toolbox 2.0: designable tunability and adaptability for 3D bioprinting applications. Small. 2022;18(25):e2106357. doi: 10.1002/smll.202106357
- Billiet T, Gevaert E, De Schryver T, Cornelissen M, Dubruel P. The 3D printing of gelatin methacrylamide cell-laden tissue-engineered constructs with high cell viability. Biomaterials. 2014;35(1):49-62. doi: 10.1016/j.biomaterials.2013.09.078
- Hospodiuk M, Dey M, Sosnoski D, Ozbolat IT. The bioink: a comprehensive review on bioprintable materials. Biotechnol Adv. 2017;35(2):217-239. doi: 10.1016/j.biotechadv.2016.12.006
- Gopinathan J, Noh I. Recent trends in bioinks for 3D printing. Biomater Res. 2018/04/06 2018;22(1):11. doi: 10.1186/s40824-018-0122-1
- Jammalamadaka U, Tappa K. Recent advances in biomaterials for 3D printing and tissue engineering. J Funct Biomater. 2018;9(1):22. doi: 10.3390/jfb9010022
- Huh J, Moon YW, Park J, Atala A, Yoo JJ, Lee SJ. Combinations of photoinitiator and UV absorber for cell-based digital light processing (DLP) bioprinting. Biofabrication. 2021;13(3): 034103. doi: 10.1088/1758-5090/abfd7a
- Peng X, Liu X, Yang Y, et al. Graphene oxide functionalized gelatin methacryloyl microgel for enhanced biomimetic mineralization and in situ bone repair. Int J Nanomedicine. 2023;18:6725-6741. doi: 10.2147/ijn.S433624
- Dinescu S, Ionita M, Ignat SR, Costache M, Hermenean A. Graphene oxide enhances chitosan-based 3D scaffold properties for bone tissue engineering. Int J Mol Sci. 2019;20(20):5077. doi: 10.3390/ijms20205077
- Li J, Liu X, Crook JM, Wallace GG. Development of 3D printable graphene oxide based bio-ink for cell support and tissue engineering. Front Bioeng Biotechnol. 2022;10:994776. doi: 10.3389/fbioe.2022.994776
- Wang C, Mallela J, Garapati US, et al. A chitosan-modified graphene nanogel for noninvasive controlled drug release. Nanomed Nanotechnol Biol Med. 2013;9(7):903-911. doi: 10.1016/j.nano.2013.01.003
- Zhang L, Li X, Shi C, et al. Biocompatibility and angiogenic effect of chitosan/graphene oxide hydrogel scaffolds on EPCs. Stem Cells International. 2021;2021:5594370. doi: 10.1155/2021/5594370
- Zhihui K, Min D. Application of graphene oxide-based hydrogels in bone tissue engineering. ACS Biomater Sci Eng. 2022;8(7):2849-2857. doi: 10.1021/acsbiomaterials.2c00396
- Zhou C, Liu S, Li J, et al. Collagen functionalized with graphene oxide enhanced biomimetic mineralization and in situ bone defect repair. ACS Appl Mater Interfaces. 2018;10(50):44080-44091. doi: 10.1021/acsami.8b17636
- Jeong J-T, Choi M-K, Sim Y, et al. Effect of graphene oxide ratio on the cell adhesion and growth behavior on a graphene oxide-coated silicon substrate. Sci Rep. 2016;6(1):33835. doi: 10.1038/srep33835
- Choe G, Oh S, Seok JM, Park SA, Lee JY. Graphene oxide/ alginate composites as novel bioinks for three-dimensional mesenchymal stem cell printing and bone regeneration applications. Nanoscale. 2019;11(48):23275-23285. doi: 10.1039/C9NR07643C
- Schmidleithner C, Malferrari S, Palgrave R, Bomze D, Schwentenwein M, Kalaskar DM. Application of high resolution DLP stereolithography for fabrication of tricalcium phosphate scaffolds for bone regeneration. Biomed Mater. 2019;14(4):045018. doi: 10.1088/1748-605X/ab279d
- Bhattacharyya A, Janarthanan G, Kim T, et al. Modulation of bioactive calcium phosphate micro/nanoparticle size and shape during in situ synthesis of photo-crosslinkable gelatin methacryloyl based nanocomposite hydrogels for 3D bioprinting and tissue engineering. Biomater Res. 2022;26(1):54. doi: 10.1186/s40824-022-00301-6
- Choi JB, Kim YK, Byeon SM, et al. Fabrication and characterization of biodegradable gelatin methacrylate/ biphasic calcium phosphate composite hydrogel for bone tissue engineering. Nanomaterials (Basel). 2021;11(3):617. doi: 10.3390/nano11030617
- Lee DN, Park JY, Seo YW, et al. Photo-crosslinked gelatin methacryloyl hydrogel strengthened with calcium phosphate-based nanoparticles for early healing of rabbit calvarial defects. J Periodontal Implant Sci. 2023;53(5):321-335. doi: 10.5051/jpis.2203220161
- Ren-Jie X, Jin-Jin M, Yu X, et al. A biphasic calcium phosphate/acylated methacrylate gelatin composite hydrogel promotes osteogenesis and bone repair. Connect Tissue Res. 2023;64(5):445-456. doi: 10.1080/03008207.2023.2212067
- Zhang X, Zhang H, Zhang Y, et al. 3D printed reduced graphene oxide-GelMA hybrid hydrogel scaffolds for potential neuralized bone regeneration. J Mater Chem B. 2023;11(6):1288-1301. doi: 10.1039/D2TB01979E
- Khan MUA, Razak SIA, Rehman S, Hasan A, Qureshi S, Stojanovic GM. Bioactive scaffold (sodium alginate)-g- (nHAp@SiO(2)@GO) for bone tissue engineering. Int J Biol Macromol. 2022;222(Pt A):462-472. doi: 10.1016/j.ijbiomac.2022.09.153
- Qi F, Wang C, Peng S, Shuai C, Yang W, Zhao Z. A co-dispersed nanosystem of strontium-anchored reduced graphene oxide to enhance the bioactivity and mechanical property of polymer scaffolds. Mater Chem Front. 2021;5(5): 2373-2386. doi: 10.1039/D0QM00958J
- Shuai C, Guo W, Wu P, et al. A graphene oxide-Ag co-dispersing nanosystem: dual synergistic effects on antibacterial activities and mechanical properties of polymer scaffolds. Chem Eng J. 2018;347:322-333. doi: 10.1016/j.cej.2018.04.092
- Saini G, Segaran N, Mayer JL, Saini A, Albadawi H, Oklu R. Applications of 3D bioprinting in tissue engineering and regenerative medicine. J Clin Med. 2021; 10(21):4966. doi: 10.3390/jcm10214966
- Vallet-Regí M, González-Calbet JM. Calcium phosphates as substitution of bone tissues. Progress in Solid State Chemistry. 2004;32(1):1-31. doi: 10.1016/j.progsolidstchem.2004.07.001
- Zhou B, Jiang X, Zhou X, et al. GelMA-based bioactive hydrogel scaffolds with multiple bone defect repair functions: therapeutic strategies and recent advances. Biomater Res. 2023;27(1):86. doi: 10.1186/s40824-023-00422-6
- Ginebra M-P, Espanol M, Maazouz Y, Bergez V, Pastorino D. Bioceramics and bone healing. EFORT Open Rev. 2018;3(5):173-183. doi: 10.1302/2058-5241.3.170056
- Alvarez K, Nakajima H. Metallic scaffolds for bone regeneration. Materials. 2009;2(3):790-832. doi: 10.3390/ma2030790
- Kühl J, Gorb S, Kern M, et al. Extrusion-based 3D printing of osteoinductive scaffolds with a spongiosa-inspired structure. Front Bioeng Biotechnol. 2023;11:1268049. doi: 10.3389/fbioe.2023.1268049
- Wang F, Saure LM, Schütt F, et al. Graphene oxide framework structures and coatings: impact on cell adhesion and pre-vascularization processes for bone grafts. Int J Mol Sci. 2022;23(6):3379. doi: 10.3390/ijms23063379
- Kolbe M, Xiang Z, Dohle E, Tonak M, Kirkpatrick CJ, Fuchs S. Paracrine effects influenced by cell culture medium and consequences on microvessel-like structures in cocultures of mesenchymal stem cells and outgrowth endothelial cells. Tissue Eng A. 2011;17(17–18):2199-2212. doi: 10.1089/ten.TEA.2010.0474
- Fuchs S, Hermanns MI, Kirkpatrick CJ. Retention of a differentiated endothelial phenotype by outgrowth endothelial cells isolated from human peripheral blood and expanded in long-term cultures. Cell Tissue Res. 2006;326:79-92. doi: 10.1007/s00441-006-0222-4
- Yue K, Trujillo-de Santiago G, Alvarez MM, Tamayol A, Annabi N, Khademhosseini A. Synthesis, properties, and biomedical applications of gelatin methacryloyl (GelMA) hydrogels. Biomaterials. 2015;73:254-271. doi: 10.1016/j.biomaterials.2015.08.045
- Fedorov A, Beichel R, Kalpathy-Cramer J, et al. 3D slicer as an image computing platform for the quantitative imaging network. Magn Reson Imaging. 2012;30(9):1323-1341. doi: 10.1016/j.mri.2012.05.001
- Cignoni P, Callieri M, Corsini M, Dellepiane M, Ganovelli F, Ranzuglia G. MeshLab: an Open-Source Mesh Processing Tool. The Eurographics Association. 2008; 129-136. doi : 10.2312/LocalChapterEvents/ItalChap/ItalianChapConf2008/129-136
- Choi CE, Chakraborty A, Adzija H, et al. Metal organic framework-incorporated three-dimensional (3D) bio-printable hydrogels to facilitate bone repair: preparation and in vitro bioactivity analysis. Gels. 2023;9(12):923. doi: 10.3390/gels9120923
- Hildebrand T, Laib A, Müller R, Dequeker J, Rüegsegger P. Direct three-dimensional morphometric analysis of human cancellous bone: microstructural data from spine, femur, iliac crest, and calcaneus. J Bone Miner Res. 1999;14(7):1167-1174. doi: 10.1359/jbmr.1999.14.7.1167
- Doktor T, Valach J, Kytyr D, Jiroušek O. Pore Size Distribution of Human Trabecular Bone – Comparison of Intrusion Measurements with Image Analysis. 2011:115-118.
- Lim TC, Chian KS, Leong KF. Cryogenic prototyping of chitosan scaffolds with controlled micro and macro architecture and their effect on in vivo neo-vascularization and cellular infiltration. J Biomed Mater Res A. 2010;94A(4):1303-1311. doi: 10.1002/jbm.a.32747
- Zhou K, Yu P, Shi X, et al. Hierarchically porous hydroxyapatite hybrid scaffold incorporated with reduced graphene oxide for rapid bone ingrowth and repair. ACS Nano. 2019;13(8):9595-9606. doi: 10.1021/acsnano.9b04723
- Taniguchi N, Fujibayashi S, Takemoto M, et al. Effect of pore size on bone ingrowth into porous titanium implants fabricated by additive manufacturing: an in vivo experiment. Mater Sci Eng C. 2016;59:690-701. doi: 10.1016/j.msec.2015.10.069
- Xia P, Luo Y. Vascularization in tissue engineering: the architecture cues of pores in scaffolds. J Biomed Mater Res B Appl Biomater. 2022;110(5):1206-1214. doi: 10.1002/jbm.b.34979
- Abdollahiyan P, Oroojalian F, Mokhtarzadeh A, de la Guardia M. Hydrogel-Based 3D Bioprinting for bone and cartilage tissue engineering. Biotechnol J. 2020;15(12):e2000095. doi: 10.1002/biot.202000095
- de Leeuw AM, Graf R, Lim PJ, et al. Physiological cell bioprinting density in human bone-derived cell-laden scaffolds enhances matrix mineralization rate and stiffness under dynamic loading. Original research. Front Bioeng Biotechnol. 2024;12:1310289. doi: 10.3389/fbioe.2024.1310289
- Dhawan A, Kennedy PM, Rizk EB, Ozbolat IT. Three-dimensional bioprinting for bone and cartilage restoration in orthopaedic surgery. J Am Acad Orthop Surg. 2019;27(5):e215-e226. doi: 10.5435/jaaos-d-17-00632
- Midha S, Dalela M, Sybil D, Patra P, Mohanty S. Advances in three-dimensional bioprinting of bone: progress and challenges. J Tissue Eng Regen Med. 2019;13(6):925-945. doi: 10.1002/term.2847
- Zhang J, Eyisoylu H, Qin X-H, Rubert M, Müller R. 3D bioprinting of graphene oxide-incorporated cell-laden bone mimicking scaffolds for promoting scaffold fidelity, osteogenic differentiation and mineralization. Acta Biomaterialia. 2021;121:637-652. doi: 10.1016/j.actbio.2020.12.026
- Chen YC, Lin RZ, Qi H, et al. Functional human vascular network generated in photocrosslinkable gelatin methacrylate hydrogels. Adv Funct Mater. 2012;22(10):2027-2039. doi: 10.1002/adfm.201101662
- Tigner TJ, Rajput S, Gaharwar AK, Alge DL. Comparison of photo cross linkable gelatin derivatives and initiators for three-dimensional extrusion bioprinting. Biomacromolecules. 2020;21(2):454-463. doi: 10.1021/acs.biomac.9b01204
- Im G-B, Lin R-Z. Bioengineering for vascularization: trends and directions of photocrosslinkable gelatin methacrylate hydrogels. Review. Front Bioeng Biotechnol. 2022;10: 1053491. doi: 10.3389/fbioe.2022.1053491
- Ahmed J, Mulla M, Maniruzzaman M. Rheological and dielectric behavior of 3D-printable chitosan/graphene oxide hydrogels. ACS Biomater Sci Eng. 2020;6(1):88-99. doi: 10.1021/acsbiomaterials.9b00201
- Kim J, Raja N, Choi YJ, et al. Enhancement of properties of a cell-laden GelMA hydrogel-based bioink via calcium phosphate phase transition. Biofabrication. 2023;16(1):ad05e2. doi: 10.1088/1758-5090/ad05e2
- Montelongo SA, Chiou G, Ong JL, Bizios R, Guda T. Development of bioinks for 3D printing microporous, sintered calcium phosphate scaffolds. J Mater Sci Mater Med. 2021;32(8):94. doi: 10.1007/s10856-021-06569-9
- Fischetti T, Borciani G, Avnet S, et al. Incorporation/ enrichment of 3D bioprinted constructs by biomimetic nanoparticles: tuning printability and cell behavior in bone models. Nanomaterials (Basel). 2023;13(14):2040. doi: 10.3390/nano13142040
- Raghav PK, Mann Z, Ahlawat S, Mohanty S. Mesenchymal stem cell-based nanoparticles and scaffolds in regenerative medicine. Eur J Pharmacol. 2022;918:174657. doi: 10.1016/j.ejphar.2021.174657
- Cernencu AI, Vlasceanu GM, Serafim A, Pircalabioru G, Ionita M. 3D double-reinforced graphene oxide – nanocellulose biomaterial inks for tissue engineered constructs. RSC Adv. 2023;13(34):24053-24063. doi: 10.1039/D3RA02786D
- Steward AJ, Kelly DJ. Mechanical regulation of mesenchymal stem cell differentiation. J Anat. 2015;227(6):717-731. doi: 10.1111/joa.12243
- Phinney DG. Functional heterogeneity of mesenchymal stem cells: implications for cell therapy. J Cell Biochem. 2012;113(9):2806-2812. doi: 10.1002/jcb.24166
- Costa LA, Eiro N, Fraile M, et al. Functional heterogeneity of mesenchymal stem cells from natural niches to culture conditions: implications for further clinical uses. Cell Mol Life Sci. 2021;78(2):447-467. doi: 10.1007/s00018-020-03600-0
- Talukdar Y, Rashkow J, Lalwani G, Kanakia S, Sitharaman B. The effects of graphene nanostructures on mesenchymal stem cells. Biomaterials. 2014;35(18):4863-4877. doi: 10.1016/j.biomaterials.2014.02.054
- Golub EE, Boesze-Battaglia K. The role of alkaline phosphatase in mineralization. Curr Opin Orthopaed. 2007;18(5):444-448. doi: 10.1097/BCO.0b013e3282630851
- Sharma U, Pal D, Prasad R. Alkaline phosphatase: an overview. Indian J Clin Biochem. 2014;29(3):269-278. doi: 10.1007/s12291-013-0408-y
- Rutkovskiy A, Stensløkken KO, Vaage IJ. Osteoblast differentiation at a glance. Med Sci Monit Basic Res. 2016;22:95-106. doi: 10.12659/msmbr.901142
- Ma H, Feng C, Chang J, Wu C. 3D-printed bioceramic scaffolds: from bone tissue engineering to tumor therapy. Acta Biomaterialia. 2018;79:37-59. doi: 10.1016/j.actbio.2018.08.026
- Wu M, Zou L, Jiang L, Zhao Z, Liu J. Osteoinductive and antimicrobial mechanisms of graphene-based materials for enhancing bone tissue engineering. J Tissue Eng Regen Med. 2021;15(11):915-935. doi: 10.1002/term.3239
- Shin SR, Li YC, Jang HL, et al. Graphene-based materials for tissue engineering. Adv Drug Deliv Rev. 2016; 105(Pt B):255-274. doi: 10.1016/j.addr.2016.03.007
- Farshid B, Lalwani G, Mohammadi MS, et al. Two-dimensional graphene oxide-reinforced porous biodegradable polymeric nanocomposites for bone tissue engineering. J Biomed Mater Res A. 2019;107(6):1143-1153. doi: 10.1002/jbm.a.36606
- Catoira MC, Fusaro L, Di Francesco D, Ramella M, Boccafoschi F. Overview of natural hydrogels for regenerative medicine applications. J Mater Sci Mater Med. 2019;30(10):115. doi: 10.1007/s10856-019-6318-7
- Zhou L, Tan G, Tan Y, Wang H, Liao J, Ning C. Biomimetic mineralization of anionic gelatin hydrogels: Effect of degree of methacrylation. RSC Adv. 2014;4:21997. doi: 10.1039/c4ra02271h
- Castillo Diaz LA, Saiani A, Gough JE, Miller AF. Human osteoblasts within soft peptide hydrogels promote mineralisation in vitro. J Tissue Eng. 2014;5:2041731414539344. doi: 10.1177/2041731414539344
- Gerhardt LC, Boccaccini AR. Bioactive glass and glass-ceramic scaffolds for bone tissue engineering. Materials. 2010;3(7):3867-3910. doi: 10.3390/ma3073867
- Morgan EF, Unnikrisnan GU, Hussein AI. Bone mechanical properties in healthy and diseased states. Annu Rev Biomed Eng. 2018;20(2018):119-143. doi: 10.1146/annurev-bioeng-062117-121139
- Diaz-Rodriguez P, Sánchez M, Landin M. Drug-loaded biomimetic ceramics for tissue engineering. Pharmaceutics. 2018;10(4):272. doi: 10.3390/pharmaceutics10040272