3D-printed Mg-substituted hydroxyapatite/ gelatin methacryloyl hydrogels encapsulated with PDA@DOX particles for bone tumor therapy and bone tissue regeneration
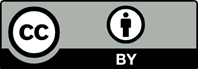
The development of bifunctional scaffolds for clinical applications, aimed at preventing tumor recurrence and promoting bone tissue regeneration simultaneously at the surgical site, is imperative in repairing bone tumor-related defects. In the current study, Mg-substituted hydroxyapatite (MgHAp) nanocomposites were synthesized via a biomineralization process. Doxorubicin hydrochloride (DOX), an anticancer drug, was incorporated in polydopamine (PDA) particles to synthesize PDA@DOX particles. MgHAp/gelatin methacryloyl (GelMA) hydrogels encapsulated with PDA@DOX particles were designed and fabricated to construct MgHAp/GelMA-PDA@DOX hydrogels via 3D printing. The 3D-printed MgHAp/GelMA-PDA@DOX hydrogels exhibited antitumor synergy by providing combined chemotherapy and phototherapy for bone tumor cell ablation. The hydrogels showed a good photothermal effect and could induce hyperthermia upon irradiation with an 808 nm near-infrared (NIR) laser. Moreover, MgHAp/GelMA-PDA@DOX hydrogels could release DOX sustainably and controllably. In vitro experiments demonstrated that 3D-printed MgHAp/GelMA-PDA@DOX hydrogels could effectively eradicate MG63 cells through the synergy of induced hyperthermia and DOX release. Furthermore, due to the sustained release of Mg2+, 3D-printed MgHAp/GelMA-PDA@DOX hydrogels could promote the proliferation of rat bone marrow-derived mesenchymal stem cells and facilitate alkaline phosphatase activity and the expression of osteogenic genes, such as osteocalcin (Ocn), type I collagen (Col1), runt-related transcription factor-2 (Runx2), and bone morphogenetic protein-2 (Bmp2), indicating their excellent osteogenic effect. As a result, 3D-printed MgHAp/GelMA-PDA@DOX hydrogels showed great potential in the treatment of bone tumor-related defects by effectively killing tumor cells and simultaneously promoting bone tissue regeneration.

- Isakoff MS, Bielack SS, Meltzer P, Gorlick R. Osteosarcoma: current treatment and a collaborative pathway to success. J Clin Oncol. 2015;33(27):3029-3035. doi: 10.1200/JCO.2014.59.4895
- Chen HHW, Kuo MT. Improving radiotherapy in cancer treatment: promises and challenges. Oncotarget. 2017;8(37):62742-62758. doi: 10.18632/oncotarget.18409
- Behranvand N, Nasri F, Zolfaghari Emameh R, et al. Chemotherapy: a double-edged sword in cancer treatment. Cancer Immunol Immunother. 2022;71(3):507-526. doi: 10.1007/s00262-021-03013-3
- Su J, Sun H, Meng Q, et al. Bioinspired nanoparticles with NIR‐controlled drug release for synergetic chemophotothermal therapy of metastatic breast cancer. Adv Funct Mater. 2016;26(41):7495-7506. doi: 10.1002/adfm.201603381
- Fan R, Chen C, Hou H, et al. Tumor acidity and near‐infrared light responsive dual drug delivery polydopamine‐based nanoparticles for chemo‐photothermal therapy. Adv Funct Mater. 2021;31(18):2009733. doi: 10.1002/adfm.202009733
- An D, Fu J, Zhang B, et al. NIR‐II responsive inorganic 2D nanomaterials for cancer photothermal therapy: recent advances and future challenges. Adv Funct Mater. 2021;31(32):2101625. doi: 10.1002/adfm.202101625
- Ren Y, Yan Y, Qi H. Photothermal conversion and transfer in photothermal therapy: from macroscale to nanoscale. Adv Colloid Interface Sci. 2022;308:102753. doi: 10.1016/j.cis.2022.102753
- Batul R, Tamanna T, Khaliq A, Yu A. Recent progress in the biomedical applications of polydopamine nanostructures. Biomater Sci. 2017;5(7):1204-1229. doi: 10.1039/c7bm00187h
- Qiu J, Shi Y, Xia Y. Polydopamine nanobottles with photothermal capability for controlled release and related applications. Adv Mater. 2021;33(45):e2104729. doi: 10.1002/adma.202104729
- Bedhiafi T, Idoudi S, Alhams AA, et al. Applications of polydopaminic nanomaterials in mucosal drug delivery. J Control Release. 2023;353:842-849. doi: 10.1016/j.jconrel.2022.12.037
- Chen S, Tan S, Zheng L, Wang M. Multilayered shape-morphing scaffolds with a hierarchical structure for uterine tissue regeneration. ACS Appl Mater Interfaces. 2024;16(6):6772-6788. doi: 10.1021/acsami.3c14983
- Abbasi N, Hamlet S, Love RM, Nguyen NT. Porous scaffolds for bone regeneration. J Sci: Adv Mater Devices. 2020; 5(1):1-9. doi: 10.1016/j.jsamd.2020.01.007
- Chen S, Shi Y, Zhang X, Ma J. Evaluation of BMP-2 and VEGF loaded 3D printed hydroxyapatite composite scaffolds with enhanced osteogenic capacity in vitro and in vivo. Mater Sci Eng C Mater Biol Appl. 2020;112:110893. doi: 10.1016/j.msec.2020.110893
- Bose S, Roy M, Bandyopadhyay A. Recent advances in bone tissue engineering scaffolds. Trends Biotechnol. 2012;30(10):546-554. doi: 10.1016/j.tibtech.2012.07.005
- Roseti L, Parisi V, Petretta M, et al. Scaffolds for bone tissue engineering: state of the art and new perspectives. Mater Sci Eng C Mater Biol Appl. 2017;78:1246-1262. doi: 10.1016/j.msec.2017.05.017
- Lai J, Wang C, Liu J, et al. Low temperature hybrid 3D printing of hierarchically porous bone tissue engineering scaffolds within situdelivery of osteogenic peptide and mesenchymal stem cells. Biofabrication. 2022;14(4):045006. doi: 10.1088/1758-5090/ac84b0
- Do AV, Khorsand B, Geary SM, Salem AK. 3D printing of scaffolds for tissue regeneration applications. Adv Healthc Mater. 2015;4(12):1742-1762. doi: 10.1002/adhm.201500168
- Wang S, Zhao S, Yu J, Gu Z, Zhang Y. Advances in translational 3D printing for cartilage, bone, and osteochondral tissue engineering. Small. 2022;18(36):e2201869. doi: 10.1002/smll.202201869
- MacDonald E, Wicker R. Multiprocess 3D printing for increasing component functionality. Science. 2016;353(6307):aaf2093. doi: 10.1126/science.aaf2093
- Velasquez-Garcia LF, Kornbluth Y. Biomedical applications of metal 3D printing. Annu Rev Biomed Eng. 2021;23: 307-338. doi: 10.1146/annurev-bioeng-082020-032402
- Ratheesh G, Vaquette C, Xiao Y. Patient-specific bone particles bioprinting for bone tissue engineering. Adv Healthc Mater. 2020;9(23):e2001323. doi: 10.1002/adhm.202001323
- Van hede D, Liang B, Anania S, et al. 3D‐printed synthetic hydroxyapatite scaffold with in silico optimized macrostructure enhances bone formation in vivo. Adv Funct Mater. 2021;32(6):2105002. doi: 10.1002/adfm.202105002
- Wang Y, Chen SS, Liang HW, Liu Y, Bai JM, Wang M. Digital light processing (DLP) of nano biphasic calcium phosphate bioceramic for making bone tissue engineering scaffolds. Ceram Int. 2022;48(19):27681-27692. doi: 10.1016/j.ceramint.2022.06.067
- Yin J, Yan M, Wang Y, Fu J, Suo H. 3D bioprinting of low-concentration cell-laden gelatin methacrylate (GelMA) bioinks with a two-step cross-linking strategy. ACS Appl Mater Interfaces. 2018;10(8):6849-6857. doi: 10.1021/acsami.7b16059
- Guo A, Zhang S, Yang R, Sui C. Enhancing the mechanical strength of 3D printed GelMA for soft tissue engineering applications. Mater Today Bio. 2024; 24:100939. doi: 10.1016/j.mtbio.2023.100939
- Chen S, Wang Y, Lai J, Tan S, Wang M. Structure and properties of gelatin methacryloyl (GelMA) synthesized in different reaction systems. Biomacromolecules. 2023;24(6):2928-2941. doi: 10.1021/acs.biomac.3c00302
- Jiang G, Li S, Yu K, et al. A 3D-printed PRP-GelMA hydrogel promotes osteochondral regeneration through M2 macrophage polarization in a rabbit model. Acta Biomater. 2021;128:150-162. doi: 10.1016/j.actbio.2021.04.010
- Xu C, Chang Y, Xu Y, et al. Silicon-phosphorus-nanosheets-integrated 3D-printable hydrogel as a bioactive and biodegradable scaffold for vascularized bone regeneration. Adv Healthc Mater. 2022;11(6):e2101911. doi: 10.1002/adhm.202101911
- Zhang X, Zhang H, Zhang Y, et al. 3D printed reduced graphene oxide-GelMA hybrid hydrogel scaffolds for potential neuralized bone regeneration. J Mater Chem B. 2023;11(6):1288-1301. doi: 10.1039/d2tb01979e
- Xavier Mendes A, Moraes Silva S, O’Connell CD, et al. Enhanced electroactivity, mechanical properties, and printability through the addition of graphene oxide to photo-cross-linkable gelatin methacryloyl hydrogel. ACS Biomater Sci Eng. 2021;7(6):2279-2295. doi: 10.1021/acsbiomaterials.0c01734
- Choi E, Kim D, Kang D, et al. 3D-printed gelatin methacrylate (GelMA)/silanated silica scaffold assisted by two-stage cooling system for hard tissue regeneration. Regen Biomater. 2021;8(2):rbab001. doi: 10.1093/rb/rbab001
- Gui XY, Zhang BQ, Song P, et al. 3D printing of biomimetic hierarchical porous architecture scaffold with dual osteoinduction and osteoconduction biofunctions for large size bone defect repair. Appl Mater Today. 2024; 37:102085. doi: 10.1016/j.apmt.2024.102085
- Pu X, Tong L, Wang X, et al. Bioinspired hydrogel anchoring 3DP GelMA/HAp scaffolds accelerates bone reconstruction. ACS Appl Mater Interfaces. 2022;14(18):20591-20602. doi: 10.1021/acsami.1c25015
- Song P, Li MX, Zhang BQ, et al. DLP fabricating of precision GelMA/HAp porous composite scaffold for bone tissue engineering application. Compos B Eng. 2022;244:110163. doi: 10.1016/j.compositesb.2022.110163
- Chen S, Shi Y, Zhang X, Ma J. Biomimetic synthesis of Mg-substituted hydroxyapatite nanocomposites and three-dimensional printing of composite scaffolds for bone regeneration. J Biomed Mater Res A. 2019;107(11): 2512-2521. doi: 10.1002/jbm.a.36757
- Zhou H, Liang B, Jiang HT, Deng ZL, Yu KX. Magnesium-based biomaterials as emerging agents for bone repair and regeneration: from mechanism to application. J Magnes Alloy. 2021;9(3):779-804. doi: 10.1016/j.jma.2021.03.004
- Chen S, Wang Y, Zhang X, Ma J, Wang M. Double-crosslinked bifunctional hydrogels with encapsulated anti-cancer drug for bone tumor cell ablation and bone tissue regeneration. Colloids Surf B Biointerfaces. 2022;213:112364. doi: 10.1016/j.colsurfb.2022.112364
- Ouyang L, Yao R, Zhao Y, Sun W. Effect of bioink properties on printability and cell viability for 3D bioplotting of embryonic stem cells. Biofabrication. 2016;8(3):035020. doi: 10.1088/1758-5090/8/3/035020
- Ma J, Wang J, Ai X, Zhang S. Biomimetic self-assembly of apatite hybrid materials: from a single molecular template to bi-/multi-molecular templates. Biotechnol Adv. 2014;32(4):744-60. doi: 10.1016/j.biotechadv.2013.10.014
- Luo Y, Chen S, Shi Y, Ma J. 3D printing of strontium-doped hydroxyapatite based composite scaffolds for repairing critical-sized rabbit calvarial defects. Biomed Mater. 2018;13(6):065004. doi: 10.1088/1748-605X/aad923
- Kang Y, Xu C, Meng L, Dong X, Qi M, Jiang D. Exosome-functionalized magnesium-organic framework-based scaffolds with osteogenic, angiogenic and anti-inflammatory properties for accelerated bone regeneration. Bioact Mater. 2022;18:26-41. doi: 10.1016/j.bioactmat.2022.02.012
- Antoniac IV, Antoniac A, Vasile E, et al. In vitro characterization of novel nanostructured collagen-hydroxyapatite composite scaffolds doped with magnesium with improved biodegradation rate for hard tissue regeneration. Bioact Mater. 2021;6(10):3383-3395. doi: 10.1016/j.bioactmat.2021.02.030
- Chen SS, Li JZ, Zheng LW, Huang J, Wang M. Biomimicking trilayer scaffolds with controlled estradiol release for uterine tissue regeneration. Exploration. 2024:20230141. doi: 10.1002/Exp.20230141
- Kumar H, Sakthivel K, Mohamed MGA, Boras E, Shin SR, Kim K. Designing gelatin methacryloyl (GelMA)-based bioinks for visible light stereolithographic 3D biofabrication. Macromol Biosci. 2021;21(1):e2000317. doi: 10.1002/mabi.202000317
- Lee BH, Lum N, Seow LY, Lim PQ, Tan LP. Synthesis and characterization of types A and B gelatin methacryloyl for bioink applications. Materials (Basel). 2016;9(10):797. doi: 10.3390/ma9100797
- Wang Z, Duan Y, Duan Y. Application of polydopamine in tumor targeted drug delivery system and its drug release behavior. J Control Release. 2018;290:56-74. doi: 10.1016/j.jconrel.2018.10.009
- Yue K, Trujillo-de Santiago G, Alvarez MM, Tamayol A, Annabi N, Khademhosseini A. Synthesis, properties, and biomedical applications of gelatin methacryloyl (GelMA) hydrogels. Biomaterials. 2015;73:254-271. doi: 10.1016/j.biomaterials.2015.08.045
- Outrequin TCR, Gamonpilas C, Siriwatwechakul W, Sreearunothai P. Extrusion-based 3D printing of food biopolymers: a highlight on the important rheological parameters to reach printability. J Food Eng. 2023; 342:111371. doi: 10.1016/j.jfoodeng.2022.111371
- Sanchez-Sanchez R, Rodriguez-Rego JM, Macias-Garcia A, Mendoza-Cerezo L, Diaz-Parralejo A. Relationship between shear-thinning rheological properties of bioinks and bioprinting parameters. Int J Bioprint. 2023; 9(2):687. doi: 10.18063/ijb.687
- Kyle S, Jessop ZM, Al-Sabah A, Whitaker IS. ‘Printability’ of candidate biomaterials for extrusion based 3D printing: state-of-the-art. Adv Healthc Mater. 2017;6(16):1700264. doi: 10.1002/adhm.201700264
- Schwab A, Levato R, D’Este M, Piluso S, Eglin D, Malda J. Printability and shape fidelity of bioinks in 3D bioprinting. Chem Rev. 2020;120(19):11028-11055. doi: 10.1021/acs.chemrev.0c00084
- Ning L, Mehta R, Cao C, et al. Embedded 3D bioprinting of gelatin methacryloyl-based constructs with highly tunable structural fidelity. ACS Appl Mater Interfaces. 2020;12(40):44563-44577. doi: 10.1021/acsami.0c15078
- Mora-Boza A, Wlodarczyk-Biegun MK, Del Campo A, Vazquez-Lasa B, Roman JS. Glycerylphytate as an ionic crosslinker for 3D printing of multi-layered scaffolds with improved shape fidelity and biological features. Biomater Sci. 2019;8(1):506-516. doi: 10.1039/c9bm01271k
- Latif M, Jiang Y, Kumar B, Song JM, Cho HC, Kim J. High content nanocellulose 3D‐printed and esterified structures with strong interfacial adhesion, high mechanical properties, and shape fidelity. Adv Mater Interfaces. 2022;9(16):202200280. doi: 10.1002/admi.202200280
- Bose S, Vahabzadeh S, Bandyopadhyay A. Bone tissue engineering using 3D printing. Mater Today. 2013;16(12):496-504. doi: 10.1016/j.mattod.2013.11.017
- Wang ZC, Huang CZ, Han X, et al. Fabrication of aerogel scaffolds with adjustable macro/micro-pore structure through 3D printing and sacrificial template method for tissue engineering. Mater Design. 2022;217(1):110662. doi: 10.1016/j.matdes.2022.110662
- Rizwan M, Chan SW, Comeau PA, Willett TL, Yim EKF. Effect of sterilization treatment on mechanical properties, biodegradation, bioactivity and printability of GelMA hydrogels. Biomed Mater. 2020;15(6):065017. doi: 10.1088/1748-605X/aba40c
- O’Connell CD, Zhang B, Onofrillo C, et al. Tailoring the mechanical properties of gelatin methacryloyl hydrogels through manipulation of the photocrosslinking conditions. Soft Matter. 2018;14(11):2142-2151. doi: 10.1039/c7sm02187a
- Bashir S, Hina M, Iqbal J, et al. Fundamental concepts of hydrogels: synthesis, properties, and their applications. Polymers (Basel). 2020;12(11):2702. doi: 10.3390/polym12112702
- Piao Y, You H, Xu T, et al. Biomedical applications of gelatin methacryloyl hydrogels. Eng Regen. 2021;2:47-56. doi: 10.1016/j.engreg.2021.03.002
- Zhang XA, Wei H, Dong C, et al. 3D printed hydrogel/ bioceramics core/shell scaffold with NIR-II triggered drug release for chemo-photothermal therapy of bone tumors and enhanced bone repair. Chem Eng J. 2023; 461:141855. doi: 10.1016/j.cej.2023.141855
- Jin A, Wang Y, Lin K, Jiang L. Nanoparticles modified by polydopamine: working as “drug” carriers. Bioact Mater. 2020;5(3):522-541. doi: 10.1016/j.bioactmat.2020.04.003
- Cheng W, Nie JP, Gao NS, et al. A multifunctional nanoplatform against multidrug resistant cancer: merging the best of targeted chemo/gene/photothermal therapy. Adv Funct Mater. 2017;27(45):1704135. doi: 10.1002/adfm.201704135
- Shahzadi I, Islam M, Saeed H, et al. Formation of biocompatible MgO/cellulose grafted hydrogel for efficient bactericidal and controlled release of doxorubicin. Int J Biol Macromol. 2022;220:1277-1286. doi: 10.1016/j.ijbiomac.2022.08.142
- Chu X, Zhang L, Li Y, He Y, Zhang Y, Du C. NIR responsive doxorubicin-loaded hollow copper ferrite @ polydopamine for dynergistic chemodynamic/photothermal/chemo-therapy. Small. 2023;19(7):e2205414. doi: 10.1002/smll.202205414
- Gao P, Fan B, Yu X, et al. Biofunctional magnesium coated Ti6Al4V scaffold enhances osteogenesis and angiogenesis in vitro and in vivo for orthopedic application. Bioact Mater. 2020;5(3):680-693. doi: 10.1016/j.bioactmat.2020.04.019
- Qian Y, Zhao X, Han Q, Chen W, Li H, Yuan W. An integrated multi-layer 3D-fabrication of PDA/RGD coated graphene loaded PCL nanoscaffold for peripheral nerve restoration. Nat Commun. 2018;9(1):323. doi: 10.1038/s41467-017-02598-7
- Yang Z, Si J, Cui Z, et al. Biomimetic composite scaffolds based on surface modification of polydopamine on electrospun poly(lactic acid)/cellulose nanofibrils. Carbohydr Polym. 2017;174:750-759. doi: 10.1016/j.carbpol.2017.07.010
- Cheng H, Chabok R, Guan X, et al. Synergistic interplay between the two major bone minerals, hydroxyapatite and whitlockite nanoparticles, for osteogenic differentiation of mesenchymal stem cells. Acta Biomater. 2018;69:342-351. doi: 10.1016/j.actbio.2018.01.016
- Liu X, Wei Y, Xuan C, et al. A biomimetic biphasic osteochondral scaffold with layer-specific release of stem cell differentiation inducers for the reconstruction of osteochondral defects. Adv Healthc Mater. 2020;9(23):e2000076. doi: 10.1002/adhm.202000076
- Li D, Zhang D, Yuan Q, et al. In vitro and in vivo assessment of the effect of biodegradable magnesium alloys on osteogenesis. Acta Biomater. 2022;141:454-465. doi: 10.1016/j.actbio.2021.12.032
- Wang L, Pang Y, Tang Y, et al. A biomimetic piezoelectric scaffold with sustained Mg(2+) release promotes neurogenic and angiogenic differentiation for enhanced bone regeneration. Bioact Mater. 2023;25:399-414. doi: 10.1016/j.bioactmat.2022.11.004
- Sun M, Liu A, Shao H, et al. Systematical evaluation of mechanically strong 3D printed diluted magnesium doping wollastonite scaffolds on osteogenic capacity in rabbit calvarial defects. Sci Rep. 2016;6:34029. doi: 10.1038/srep34029