Manufacturing and degrading features of 3D-printed porous spinal interbody fusion cages
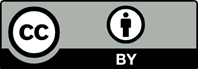
Spinal fusion operations are often utilized to address disc degeneration, vertebral slippage, instability, and trauma, and interbody fusion cages have been widely employed in these procedures. The fundamental aim of an interbody fusion cage is to give immediate interbody support, height, and biomechanical stability of the spinal space to enable bone development in the fused area. With the aim to address shortcomings of the currently commonly used clinical spinal interbody fusion cages, such as non-osteogenic activity, non-resorbability, biomechanical mismatch, etc., composites made of polycaprolactone (PCL) were prepared in this study, with the addition of hydroxyapatite (HA) that possesses both osteoinductive properties and enhanced mechanical strength as a functional filler. An innovative bi-directional variable meso-structure scheme is proposed. The porous degradable spinal interbody fusion cage was manufactured by using polymer melt differential three-dimensional (3D) printing technology. The study of the cage’s 3D structural characteristics on the degradation properties and the influence of the degradation process on its mechanical properties was carried out. Preliminary cell viability assays were also conducted. This study showed that the compressive strength of the cages increases with the aperture diameter and the number of crossing layers of the beams, and the compressive modulus is positively associated with the number of crossing layers of the beams. The degradation rate of the cage grew with the reduction of its filling rate and the rise of the number of crossing layers of the beams, i.e., the degradation rate increased with the expansion of the internal aperture. The cage with a 60% internal filling rate and containing 1 or 2 crossing layers of beams is more suited for spinal fusion, and with a pore size between 450 and 490 μm, the fundamental structure of the cage can be preserved while maintaining strong support performance throughout degradation. In addition, the 3D printing process in this study does not cause an increase in cytotoxicity, making it a feasible bioprinting method.
- Verma R, Virk S, Qureshi S. Interbody fusions in the lumbar spine: a review. HSS J. 2020;16(2):162-167. doi: 10.1007/s11420-019-09737-4
- Mobbs RJ, Phan K, Malham G, Seex K, Rao PJ. Lumbar interbody fusion: techniques, indications and comparison of interbody fusion options including PLIF, TLIF, MI-TLIF, OLIF/ATP, LLIF and ALIF. J Spine Surg. 2015;1(1):2-18. doi: 10.3978/j.issn.2414-469X.2015.10.05
- Vaccaro AR, Chiba K, Heller JG, et al. Bone grafting alternatives in spinal surgery. Spine J. 2002;2(3):206-215. doi: 10.1016/S1529-9430(02)00180-8
- Liu S, Wang Y, Liang Z, Zhou M, Chen C. Comparative clinical effectiveness and safety of bone morphogenetic protein versus autologous iliac crest bone graft in lumbar fusion: a meta-analysis and systematic review. Spine. 2020;45(12):E729-E741. doi: 10.1097/BRS.0000000000003372
- Geisinger JR, Park DK. Allograft bone: uses in spinal surgery. Semin Spine Surg. 2016;28(4):190-195. doi: 10.1053/j.semss.2016.08.002
- Rao PJ, Pelletier MH, Walsh WR, Mobbs RJ. Spine interbody implants: material selection and modification, functionalization and bioactivation of surfaces to improve osseointegration: bioactivation of spine interbody implant surfaces. Orthop Surg. 2014;6(2):81-89. doi: 10.1111/os.12098
- Yin X. Application of biodegradable 3D-printed cage for cervical diseases via anterior cervical discectomy and fusion (ACDF): an in vitro biomechanical study. Biotechnol Lett. 2017;39(9):1433-1439. doi: 10.1007/s10529-017-2367-5
- Chen Y, Wang X, Lu X, et al. Comparison of titanium and polyetheretherketone (PEEK) cages in the surgical treatment of multilevel cervical spondylotic myelopathy: a prospective, randomized, control study with over 7-year follow-up. Eur Spine J. 2013;22(7):1539-1546. doi: 10.1007/s00586-013-2772-y
- Karikari IO, Jain D, Owens TR, et al. Impact of subsidence on clinical outcomes and radiographic fusion rates in anterior cervical discectomy and fusion: a systematic review. J Spinal Disord Tech. 2014;27(1):1-10. doi: 10.1097/BSD.0b013e31825bd26d
- Cuzzocrea F, Ivone A, Jannelli E, et al. PEEK versus metal cages in posterior lumbar interbody fusion: a clinical and radiological comparative study. Musculoskelet Surg. 2019;103(3):237-241. doi: 10.1007/s12306-018-0580-6
- Hasegawa T, Ushirozako H, Shigeto E, et al. The titanium-coated PEEK cage maintains better bone fusion with the endplate than the PEEK cage 6 months after PLIF surgery: a multicenter, prospective, randomized study. Spine. 2020;45(15):E892-E902. doi: 10.1097/BRS.0000000000003464
- Laubach M, Kobbe P, Hutmacher DW. Biodegradable interbody cages for lumbar spine fusion: current concepts and future directions. Biomaterials. 2022;288:121699. doi: 10.1016/j.biomaterials.2022.121699
- Koutserimpas C, Alpantaki K, Chatzinikolaidou M, Chlouverakis G, Dohm M, Hadjipavlou AG. The effectiveness of biodegradable instrumentation in the treatment of spinal fractures. Injury. 2018;49(12):2111-2120. doi: 10.1016/j.injury.2018.11.008
- Daskalakis E, Liu F, Huang B, et al. Investigating the influence of architecture and material composition of 3D printed anatomical design scaffolds for large bone defects. Int J Bioprint. 2021;7(2):268. doi: 10.18063/ijb.v7i2.268
- Han X, Gao Y, Ding Y, et al. In vitro performance of 3D printed PCL −β -TCP degradable spinal fusion cage. J Biomater Appl. 2021;35(10):1304-1314. doi: 10.1177/0885328220978492
- Abbah SA, Lam CXF, Ramruttun AK, Goh JCH, Wong HK. Fusion performance of low-dose recombinant human bone morphogenetic protein 2 and bone marrow-derived multipotent stromal cells in biodegradable scaffolds. Spine. 2011;36(21):1752-1759. doi: 10.1097/BRS.0b013e31822576a4
- Cao L, Chen Q, Jiang L-B, et al. Bioabsorbable self-retaining PLA/nano-sized beta-TCP cervical spine interbody fusion cage in goat models: an in vivo study. IJN. 2017;12:7197-7205. doi: 10.2147/IJN.S132041
- Rezania N. Three-dimensional printing of polycaprolactone/ hydroxyapatite bone tissue engineering scaffolds mechanical properties and biological behavior. J Mater Sci. 2022;33(3):31. doi: 10.1007/s10856-022-06653-8
- Liu F, Kang H, Liu Z, et al. 3D printed multi-functional scaffolds based on poly(ε-caprolactone) and hydroxyapatite composites. Nanomaterials. 2021;11(9):2456. doi: 10.3390/nano11092456
- Backes EH, Beatrice CAG, Shimomura KMB, et al. Development of poly(Ɛ-polycaprolactone)/hydroxyapatite composites for bone tissue regeneration. J Mater Res. 2021;36(15):3050-3062. doi: 10.1557/s43578-021-00316-0
- Wang F, Tankus EB, Santarella F, et al. Fabrication and characterization of PCL/HA filament as a 3D printing material using thermal extrusion technology for bone tissue engineering. Polymers. 2022;14(4):669. doi: 10.3390/polym14040669
- Ma J. Modification of 3D printed PCL scaffolds by PVAc and HA to enhance cytocompatibility and osteogenesis. RSC Adv. 2019;9(10):5338-5346. doi: 10.1039/c8ra06652c
- Doyle SE, Henry L, McGennisken E, et al. Characterization of polycaprolactone nanohydroxyapatite composites with tunable degradability suitable for indirect printing. Polymers. 2021;13(2):295. doi: 10.3390/polym13020295
- Shikinami Y, Okuno M. Mechanical evaluation of novel spinal interbody fusion cages made of bioactive, resorbable composites. Biomaterials. 2003;24(18):3161-3170. doi: 10.1016/S0142-9612(03)00155-8
- Jiao Z, Luo B, Xiang S, Ma H, Yu Y, Yang W. 3D printing of HA / PCL composite tissue engineering scaffolds. Adv Ind Eng Polym Res. 2019;2(4):196-202. doi: 10.1016/j.aiepr.2019.09.003
- Liu D, Nie W, Li D, et al. 3D printed PCL/SrHA scaffold for enhanced bone regeneration. Chem Eng J. 2019;362: 269-279. doi: 10.1016/j.cej.2019.01.015
- Kim HS, Song JS, Heo W, Cha JH, Rhee DY. Comparative study between a curved and a wedge PEEK cage for single-level anterior cervical discectomy and interbody fusion. Korean J Spine. 2012;9(3):181-186. doi: 10.14245/kjs.2012.9.3.181
- Stella JA, D’Amore A, Wagner WR, Sacks MS. On the biomechanical function of scaffolds for engineering load-bearing soft tissues. Acta Biomater. 2010;6(7):2365-2381. doi: 10.1016/j.actbio.2010.01.001
- Knutsen AR. Static and dynamic fatigue behavior of topology designed and conventional 3D printed bioresorbable PCL cervical interbody fusion devices. J Mech Behav Biomed Mater. 2015;49:332-342. doi: 10.1016/j.jmbbm.2015.05.015
- Bittner SM, Smith BT, Diaz-Gomez L, et al. Fabrication and mechanical characterization of 3D printed vertical uniform and gradient scaffolds for bone and osteochondral tissue engineering. Acta Biomater. 2019;90:37-48. doi: 10.1016/j.actbio.2019.03.041
- Zhang Y, Yu W, Ba Z, Cui S, Wei J, Li H. 3D-printed scaffolds of mesoporous bioglass/gliadin/polycaprolactone ternary composite for enhancement of compressive strength, degradability, cell responses and new bone tissue ingrowth. Int J Nanomed. 2018;13:5433-5447. doi: 10.2147/IJN.S164869
- Liu H, Ahlinder A, Yassin MA, Finne-Wistrand A, Gasser TC. Computational and experimental characterization of 3D-printed PCL structures toward the design of soft biological tissue scaffolds. Mater Des. 2020;188:11. doi: 10.1016/j.matdes.2020.108488
- Scocozza F. Shape fidelity and sterility assessment of 3D printed polycaprolactone and hydroxyapatite scaffolds. J Polym Res. 2021;28(9):327. doi: 10.1007/s10965-021-02675-y
- Kia C, Antonacci CL, Wellington I, Makanji HS, Esmende SM. Spinal implant osseointegration and the role of 3D printing: an analysis and review of the literature. Bioengineering. 2022;9(3):108. doi: 10.3390/bioengineering9030108
- Wixted CM, Peterson JR, Kadakia RJ, Adams SB. Three-dimensional printing in orthopaedic surgery: current applications and future developments. JAAOS Glob Res Rev. 2021;5(4):e20.00230-11. doi: 10.5435/JAAOSGlobal-D-20-00230
- Amelot A, Colman M, Loret J-E. Vertebral body replacement using patient-specific three–dimensional-printed polymer implants in cervical spondylotic myelopathy: an encouraging preliminary report. Spine J. 2018;18(5):892-899. doi: 10.1016/j.spinee.2018.01.019
- Pan CT, Lin CH, Huang YS, et al. Design of interbody fusion cages of Ti6Al4V with gradient porosity using a selective laser melting process for spinal fusion arthroplasty. J Laser Micro/Nanoeng. 2017;12(1):34-44. doi: 10.2961/jlmn.2017.01.0007
- Egan P, Wang X, Greutert H, Shea K, Wuertz-Kozak K, Ferguson S. Mechanical and biological characterization of 3D printed lattices. 3D Print Addit Manuf. 2019;6(2):73-81. doi: 10.1089/3dp.2018.0125
- Wang H, Li Y, Zuo Y, Li J, Ma S, Cheng L. Biocompatibility and osteogenesis of biomimetic nano-hydroxyapatite/ polyamide composite scaffolds for bone tissue engineering. Biomaterials. 2007;28(22):3338-3348. doi: 10.1016/j.biomaterials.2007.04.014
- Malikmammadov E, Tanir TE, Kiziltay A, Hasirci V, Hasirci N. PCL and PCL-based materials in biomedical applications. J Biomater Sci Polym Ed. 2018;29(7-9): 863-893. doi: 10.1080/09205063.2017.1394711
- Gibson LJ, Ashby MF. Cellular Solids: Structure and Properties. 2nd ed. Cambridge, UK: Cambridge University Press; Cambridge, UK. 1999.