Multi-physical field control piezoelectric inkjet bioprinting for 3D tissue-like structure manufacturing
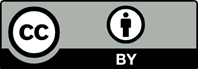
With high precision, drop-on-demand, and noncontact material delivery advantages, inkjet bioprinting technology has been widely used in tissue manufacturing. However, the main challenge of inkjet bioprinting is that the bioink must be liquid-like in the printhead to avoid clogging the nozzle, then form microdroplets, and finally undergo crosslinking to quickly form a gel and make an object with strength and precision. The primary solution relies on the fast crosslinking of sodium alginate by calcium chloride. Nevertheless, it is difficult to guarantee the precision of inkjet bioprinting with this method, and cumulative errors lead to the inability to print high aspect ratio three-dimensional (3D) structures. Additionally, sodium alginate lacks cell adhesion sites, and calcium chloride at high concentrations is toxic to cells. To solve the above problems, we present a new printing method called multi-physical field control piezoelectric inkjet bioprinting (MFCPIB) for making 3D tissue-like structures using 5% gelatin methacryloyl (GelMA). For extrusion and photocuring 3D bioprinting tasks, 5% GelMA is widely used due to its favorable biocompatibility. In this study, we accomplished a 5% GelMA inkjet bioprinting for the first time by leveraging the MFCPIB method. Our experimental results demonstrated the feasibility of this approach for printing GelMA of different concentrations. The temperature-sensitive GelMA was utilized during the printing process in which GelMA is liquid-like in the high-temperature printhead, cools in the form of microdroplets in cold air after injection, and finally photocrosslinks to form a permanent gel. We analyzed the inverse piezoelectric effect and fluid dynamics to control the pressure field, which in turn controls the velocity and diameter of the microdroplets. After conducting a simulation analysis of the temperature field and performing calculations using the lumped parameter method, we implemented a dual closed-loop control strategy to ensure precise temperature control of the microdroplets. Furthermore, based on the analysis of energy conversion, we obtained the pressure and temperature field control laws corresponding to the ideal printable temperature of the microdroplet. Using the MFCPIB method, different 3D structures were successfully printed with GelMA. For example, a cell-laden vessel-like structure with an aspect ratio of 4.0 was achieved. The proposed MFCPIB method did not influence the viability of smooth muscle cells after printing, demonstrating the potential for fabricating tissues with high bioactivity.
- Mota C, Camarero-Espinosa S, Baker MB, Wieringa P, Moroni L. Bioprinting: from tissue and organ development to in vitro models. Chem Rev. 2020;120:10547–10607. doi: 10.1021/acs.chemrev.9b00789
- Zhou L, Fu J, He Y. A review of 3D printing technologies for soft polymer materials. Adv Funct Mater. 2020;30:2000187. doi: 10.1002/adfm.202000187
- Wang H, Guo K, Zhang L, et al. Valve-based consecutive bioprinting method for multimaterial tissue-like constructs with controllable interfaces. Biofabrication. 2021;13:035001. doi: 10.1088/1758-5090/abdb86
- Guo K, Wang H, Li S, et al. Bioprinting of light-crosslinkable neutral-dissolved collagen to build implantable connective tissue with programmable cellular orientation. Biofabrication. 2023;15:035007. doi: 10.1088/1758-5090/acc760
- Blaeser A, Duarte Campos DF, Puster U, Richtering W, Stevens MM, Fischer H. Controlling shear stress in 3D bioprinting is a key factor to balance printing resolution and stem cell integrity. Adv Healthc Mater. 2016;5:326-333. doi: 10.1002/adhm.201500677
- Masaeli E, Marquette C. Direct-write bioprinting approach to construct multilayer cellular tissues. Front Bioeng Biotechnol. 2020;7:478. doi: 10.3389/fbioe.2019.00478
- Li X, Chen J, Liu B, Wang X, Ren D, Xu T. Inkjet printing for biofabrication. In: Ovsianikov A, Yoo J, Mironov V, eds. 3D Printing and Biofabrication. Cham: Springer; 2018: 283-301. doi: 10.1007/978-3-319-45444-3_26
- Zhao L, Chang Yan K, Yao R, Lin F, Sun W. Alternating force based drop-on-demand microdroplet formation and three-dimensional deposition. J Manuf Sci Eng. 2015;137:031009. doi: 10.1115/1.4029803
- Takagi D, Lin W, Matsumoto T, et al. High-precision three-dimensional inkjet technology for live cell bioprinting. Int J Bioprint. 2019;5(2):208. doi: 10.18063/ijb.v5i2.208.
- Li EQ, Xu Q, Sun J, Fuh JYH, Wong YS, Thoroddsen ST. Design and fabrication of a PET/PTFE-based piezoelectric squeeze mode drop-on-demand inkjet printhead with interchangeable nozzle. Sens Actuators Phys. 2010;163:315-322. doi: 10.1016/j.sna.2010.07.014
- Foresti D, Kroll KT, Amissah R, et al. Acoustophoretic printing. Sci Adv. 2018;4:eaat1659. doi: 10.1126/sciadv.aat1659
- Li X, Liu B, Pei B, et al. Inkjet bioprinting of biomaterials. Chem Rev. 2020;120:10793-10833. doi: 10.1021/acs.chemrev.0c00008
- Ringeisen BR, Spargo BJ, Wu PK, eds. Cell and Organ Printing. New York, NY: Springer; 2010. doi: 10.1007/978-90-481-9145-1
- Zimmermann R, Hentschel C, Schrön F, et al. High resolution bioprinting of multi-component hydrogels. Biofabrication. 2019;11:045008. doi: 10.1088/1758-5090/ab2aa1
- Christensen K, Xu C, Chai W, Zhang Z, Fu J, Huang Y. Freeform inkjet printing of cellular structures with bifurcations. Biotechnol Bioeng. 2015;112:1047-1055. doi: 10.1002/bit.25501
- Yoon S, Park JA, Lee H-R, Yoon WH, Hwang DS, Jung S. Inkjet-spray hybrid printing for 3D freeform fabrication of multilayered hydrogel structures. Adv Healthc Mater. 2018;7:1800050. doi: 10.1002/adhm.201800050
- Cheng C, Moon YJ, Kim SH, et al. Water-matrix interaction at the drop-drop interface during drop-on-demand printing of hydrogels. Int J Heat Mass Transf. 2020;150:119327. doi: 10.1016/j.ijheatmasstransfer.2020.119327
- Sakurada S, Sole-Gras M, Christensen K, Wallace DB, Huang Y. Liquid-absorbing system-assisted intersecting jets printing of soft structures from reactive biomaterials. Addit Manuf. 2020;31:100934. doi: 10.1016/j.addma.2019.100934
- Teo MY, Kee S, RaviChandran N, Stuart L, Aw KC, Stringer J. Enabling free-standing 3D hydrogel microstructures with microreactive inkjet printing. ACS Appl Mater Interfaces. 2020;12:1832-1839. doi: 10.1021/acsami.9b17192
- Guo K, Wang H, Li S, et al. Collagen-based thiol–norbornene photoclick bio-ink with excellent bioactivity and printability. ACS Appl Mater Interfaces. 2021;13:7037-7050.doi: 10.1021/acsami.0c16714
- Yue K, Trujillo-de Santiago G, Alvarez MM, Tamayol A, Annabi N, Khademhosseini A. Synthesis, properties, and biomedical applications of gelatin methacryloyl (GelMA) hydrogels. Biomaterials. 2015;73:254-271. doi: 10.1016/j.biomaterials.2015.08.045
- Zhang F, Zhang Z, Duan X, et al. Integrating zinc/silicon dual ions with 3D-printed GelMA hydrogel promotes in situ hair follicle regeneration. Int J Bioprint. 2023;9(3):703. doi: 10.18063/ijb.703
- Klotz BJ, Gawlitta D, Rosenberg AJWP, Malda J, Melchels FPW. Gelatin-methacryloyl hydrogels: towards biofabrication-based tissue repair. Trends Biotechnol. 2016;34:394-407. doi: 10.1016/j.tibtech.2016.01.002
- Sun M, You D, Zhan N, et al. 4D oriented dynamic scaffold for promoting peripheral nerve regeneration and functional recovery. Adv Funct Mater. 2023;2305827. doi: 10.1002/adfm.202305827
- Zhang J, Chen Y, Huang Y, et al. A 3D‐printed self‐adhesive bandage with drug release for peripheral nerve repair. Adv Sci. 2020;7:2002601. doi: 10.1002/advs.202002601
- Liu W, Heinrich MA, Zhou Y, et al. Extrusion bioprinting of shear‐thinning gelatin methacryloyl bioinks. Adv Healthc Mater. 2017;6:1601451. doi: 10.1002/adhm.201601451
- Ying G, Jiang N, Yu C, Zhang YS. Three-dimensional bioprinting of gelatin methacryloyl (GelMA). Bio-Des Manuf. 2018;1(4):215-224. doi: 10.1007/s42242-018-0028-8
- Zhang L, Zhang H, Wang H, et al. Fabrication of multi-channel nerve guidance conduits containing schwann cells based on multi-material 3D bioprinting. 3D Print Addit Manuf. 2022;10(5):1046-1054. doi: 10.1089/3dp.2021.0203
- Suntornnond R, Ng WL, Huang X, Yeowa CHE, Yeong WY. Improving printability of hydrogel-based bio-inks for thermal inkjet bioprinting applications via saponification and heat treatment processes. J Mater Chem B. 2022;10:5989-6000. doi: 10.1039/D2TB00442A
- Liu X, Wang X, Zhang L, et al. 3D liver tissue model with branched vascular networks by multimaterial bioprinting. Adv Healthc Mater. 2021;10:2101405. doi: 10.1002/adhm.202101405
- Shao L, Gao Q, Xie C, et al. Sacrificial microgel-laden bioink-enabled 3D bioprinting of mesoscale pore networks. Bio-Des Manuf. 2020;3:30-39. doi: 10.1007/s42242-020-00062-y
- Mazur P. Freezing of living cells: mechanisms and implications. Am J Physiol. 1984;247:C125-C142. doi: 10.1152/ajpcell.1984.247.3.C125
- Zhou J, Pei Z. Experimental study of the piezoelectric drop-on-demand drop formation in a coaxial airflow. Chem Eng Process. 2020;147:107778. doi: 10.1016/j.cep.2019.107778
- Binder KW, Allen AJ, Yoo JJ, Atala A. Drop-on-demand inkjet bioprinting: a primer. Gene Ther Regul. 2011;06: 33-49. doi: 10.1142/S1568558611000258
- Jaffe H, Berlincourt DA. Piezoelectric transducer materials. Proc IEEE. 1965;53:1372-1386. doi: 10.1109/PROC.1965.4253
- McLean D. Understanding Aerodynamics: Arguing from the Real Physics. Chichester, West Sussex: McLean John Wiley and Sons 2012. doi: 10.1002/9781118454190
- Chang H-J, Tsai MH, Hwang W-S. The simulation of micro droplet behavior of molten lead-free solder in inkjet printing process and its experimental validation. Appl Math Model. 2012;36:3067–-3079. doi: 10.1016/j.apm.2011.09.094
- He M, Sun L, Hu K, Zhu Y, Ma L, Chen H. Drop-on-demand inkjet printhead performance enhancement by dynamic lumped element modeling for printable electronics fabrication. Math Probl Eng. 2014;2014:1-16. doi: 10.1155/2014/270679
- Gallas Q, Sheplak M, Kaysap A, et al. Lumped element modeling of piezoelectric-driven synthetic jet actuators. 40th AIAA Aerospace Sciences Meeting & Exhibit. 2002. doi: 10.2514/6.2002-125
- Shah MA, Lee D-G, Lee BY, Kim NW, An H, Hur S. Actuating voltage waveform optimization of piezoelectric inkjet printhead for suppression of residual vibrations. Micromachines. 2020;11:900. doi: 2072-666X/11/10/900
- Liu H, Liu J, Qi C, et al. Thermosensitive injectable in-situ forming carboxymethyl chitin hydrogel for three-dimensional cell culture. Acta Biomater. 2016; 35:228-237. doi: 10.1016/j.actbio.2016.02.028
- Shih H, Lin C-C. Visible-light-mediated thiol-ene hydrogelation using eosin-Y as the only photoinitiator. Macromol Rapid Commun. 2013;34:269-273. doi: 10.1002/marc.201200605
- Guvendiren M, Burdick JA. Stiffening hydrogels to probe short- and long-term cellular responses to dynamic mechanics. Nat Commun. 2012;3:792. doi: 10.1038/ncomms1792
- Csemány D. Thermal analysis of suspended single droplet evaporation measurements with a coupled lumped parameter model. Heat Mass Transf. 2023;59:2181-2195. doi: 10.1007/s00231-023-03403-6
- Sazhin SS, Gol’dshtein VA, Heikal MR. A transient formulation of newton’s cooling law for spherical bodies. J Heat Transf. 2001;123:63-64. doi: 10.1115/1.1337650
- Gong Y, Bi Z, Bian X, et al. Study on linear bio-structure print process based on alginate bio-ink in 3D bio-fabrication. Bio- Des Manuf. 2020;3:109-121. doi: 10.1007/s42242-020-00065-9