A parallel multilayered neurovascular unit-on-a-chip for modeling neurovascular microenvironment and screening chemotherapeutic drugs
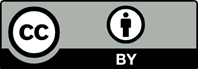
Humans are grappling with increasing occurrence rate of brain tumors, which are associated with unfavorable prognosis and cognitive neurotoxicity caused by chemotherapy. Ideally, therapeutic drugs for brain tumors should efficiently suppress the tumors while minimizing neurotoxic effects. To facilitate drug development, in vitro pathological models of human brain tumors are essential. Here, we engineered a sophisticated human neurovascular unit (hNVU) chip that simulates the microenvironment of pediatric brain using three-dimensional bioprinting technology. The hNVU chip featured a multilayer parallel architecture composed of a biomimetic blood–blood barrier (BBB) and brain region. An automated perfusion system in the chip simulated the blood flow within the brain. The specialized liquid channels within the brain region enabled comprehensive analysis of drug metabolites. The blood–brain barrier (BBB) structure is composed of endothelial cells, pericytes, and astrocytes, and the brain region contains endothelial cells, pericytes, astrocytes, microglia, and neural progenitor cells, representing the cellular components found in pediatric brain. To replicate the basal membrane, we utilized GelMA (gelatin methacryloyl), gelatin, and laminin as primary bioinks, with the addition of fibrin to mimic the pathological characteristics of brain extracellular matrix (ECM). The BBB membrane exhibited a maximum electrical resistance of 360 Ω cm2 and demonstrated impressive semipermeability by effectively blocking the permeability of large molecules with a molecular weight of 10 kDa. Gene expression analysis and immunofluorescence staining revealed a gradual increase in COL4A1 and fibrin, indicating remodeling of the ECM by the cells. Astrocytoma cells (SF188) were introduced in the model to construct a neurovascular unit containing pediatric brain tumor cells. We evaluated the therapeutic effects and toxicity profiles of chemotherapeutic drugs, including vorinostat and 5-fluorouracil. Encouragingly, our investigations revealed that vorinostat not only exhibited remarkable tumor-suppressing efficacy but also induced lower neurocognitive toxicity than 5-fluorouracil. In summary, the hNVU chip served as a reliable and versatile platform for conducting comprehensive studies on the therapeutic effects of chemotherapy drugs for brain tumors.
- Louis DN, Ohgaki H, Wiestler OD, et al. The 2007 WHO classification of tumors of the central nervous system. Acta Neuropathol. 2007;114:97-109. doi: 10.1007/s00401-007-0243-4
- Biserova K, Jakovlevs A, Uljanovs R, Strumfa I. Cancer stem cells: significance in origin, pathogenesis and treatment of glioblastoma. Cells. 2021;10(3):621. doi: 10.3390/cells10030621
- Manoranjan B, Garg N, Bakhshinyan D, Singh SK. The role of stem cells in pediatric central nervous system malignancies. Adv Exp Med Biol. 2015;853:49-68. doi: 10.1007/978-3-319-16537-0_4
- Jessa S, Blanchet-Cohen A, Krug B, et al. Stalled developmental programs at the root of pediatric brain tumors. Nat Genet. 2019;51:1702-1713. doi: 10.1038/s41588-019-0531-7
- Mackay A, Burford A, Carvalho D, et al. Integrated molecular meta-analysis of 1,000 pediatric high-grade and diffuse intrinsic pontine glioma. Cancer Cell. 2017;32(4): 520-537.e5. doi: 10.1016/j.ccell.2017.08.017
- Ray S. Tumorsphere formation assay: a cancer stem-like cell characterization in pediatric brain cancer medulloblastoma. In: Bhakat KK, Hazra TK, eds. Base Excision Repair Pathway. Methods in Molecular Biology, vol. 2701. New York, NY: Humana; 2023. doi: 10.1007/978-1-0716-3373-1_17
- Mo J, Anastasaki C, Chen Z. Humanized neurofibroma model from induced pluripotent stem cells delineates tumor pathogenesis and developmental origins. J Clin Invest. 2021;131(1):e139807. doi: 10.1172/JCI139807
- Maurange C. Temporal patterning in neural progenitors: from Drosophila development to childhood cancers. Dis Model Mech. 2020;13(7):dmm044883. doi: 10.1242/dmm.044883
- Lin S, Li K, Qi L. Cancer stem cells in brain tumors: from origin to clinical implications. MedComm. 2023;4:e341. doi: 10.1002/mco2.341
- Mascelli S, Raso A, Biassoni R, et al. Analysis of NADP+- dependent isocitrate dehydrogenase-1/2 gene mutations in pediatric brain tumors: report of a secondary anaplastic astrocytoma carrying the IDH1 mutation. J Neurooncol. 2012;109(3):477-484. doi: 10.1007/s11060-012-0925-1
- Dubuc AM, Northcott PA, Mack S, Witt H, Pfister S, Taylor MD. The genetics of pediatric brain tumors. Curr Neurol Neurosci Rep. 2010;10:215-223. doi: 10.1007/s11910-010-0103-9
- Deng MY, Sturm D, Pfaff E, et al. Radiation-induced gliomas represent H3-/IDH-wild type pediatric gliomas with recurrent PDGFRA amplification and loss of CDKN2A/B. Nat Commun. 2021;12:5530. doi: 10.1038/s41467-021-25708-y
- Kitange GJ, Carlson BL, Schroeder MA, et al. Induction of MGMT expression is associated with temozolomide resistance in glioblastoma xenografts. Neuro-Oncol. 2009,11(3):281-291. doi: 10.1215/15228517-2008-090
- Alnahhas I, Alsawas M, Rayi A, et al. Characterizing benefit from temozolomide in MGMT promoter unmethylated and methylated glioblastoma: a systematic review and meta-analysis. Neuro-Oncol Adv. 2020;3(1):vdaa082. doi: 10.1093/noajnl/vdaa082
- Verscheijden LFM, van Hattem AC, Pertijs JCLM, et al. Developmental patterns in human blood–brain barrier and blood–cerebrospinal fluid barrier ABC drug transporter expression. Histochem Cell Biol. 2020;154:265-273. doi: 10.1007/s00418-020-01884-8
- Partap S, Monje M. Pediatric brain tumors. Continuum (Minneap Minn). 2020;26:1553-1583. doi: 10.1212/CON.0000000000000955
- Leary SES, Kilburn L, Geyer JR, et al. Vorinostat and isotretinoin with chemotherapy in young children with embryonal brain tumors: a report from the Pediatric Brain Tumor Consortium (PBTC-026). Neuro Oncol. 2022;24(7):1178-1190. doi: 10.1093/neuonc/noab293
- Lam S, Lin Y, Warnke PC. Permeability imaging in pediatric brain tumors. Transl Pediatr. 2014;3(3):218-228. doi: 10.3978/j.issn.2224-4336.2014.07.01
- Langen UH, Ayloo S, Gu C. Development and cell biology of the blood‒brain barrier. Annu Rev Cell Dev Biol. 2019;35:591-613. doi: 10.1146/annurev-cellbio-100617-062608
- Arvanitis CD, Ferraro GB, Jain RK. The blood–brain barrier and blood–tumor barrier in brain tumors and metastases. Nat Rev Cancer. 2020;20:26-41. doi: 10.1038/s41568-019-0205-x
- Venneti S, Thompson CB. Metabolic reprogramming in brain tumors. Annu Rev Pathol. 2017;12:515-545. doi: 10.1146/annurev-pathol-012615-044329
- Maoz B, Herland A, FitzGerald EA, et al. A linked organ-on-chip model of the human neurovascular unit reveals the metabolic coupling of endothelial and neuronal cells. Nat Biotechnol. 2018;36:865-874. doi: 10.1038/nbt.4226
- Kim J, Lee KT, Lee JS, et al. Fungal brain infection modeled in a human-neurovascular-unit-on-a-chip with a functional blood–brain barrier. Nat Biomed Eng. 2021;5:830-846. doi: 10.1038/s41551-021-00743-8
- Ahn SI, Sei YJ, Park HJ, et al. Microengineered human blood– brain barrier platform for understanding nanoparticle transport mechanisms. Nat Commun. 2020;11:175. doi: 10.1038/s41467-019-13896-7
- Lyu Z, Park J, Kim KM, et al. A neurovascular-unit-on-a-chip for the evaluation of the restorative potential of stem cell therapies for ischemic stroke. Nat Biomed Eng. 2021;5:847-863. doi: 10.1038/s41551-021-00744-7
- Ouyang L, Armstrong JPK, Chen Q, Lin Y, Stevens MM. Void-free 3D bioprinting for in situ endothelialization and microfluidic perfusion. Adv Funct Mater. 2020; 30:1908349. doi: 10.1002/adfm.201908349
- Cho WW, Ahn M, Kim BS, Cho DW. Blood-lymphatic integrated system with heterogeneous melanoma spheroids via in-bath three-dimensional bioprinting for modeling of combinational targeted therapy. Adv Sci. 2022;9(29):e2202093. doi: 10.1002/advs.202202093
- Potjewyd G, Moxon S, Wang T, Domingos M, Hooper NM. Tissue engineering 3D neurovascular units: a biomaterials and bioprinting perspective. Trends Biotechnol. 2018;36(4):457-472. doi: 10.1016/j.tibtech.2018.01.003
- Neufeld L, Yeini E, Reisman N, et al. Microengineered perfusable 3D-bioprinted glioblastoma model for in vivo mimicry of tumor microenvironment. Sci Adv. 2021;7(34):eabi9119. doi: 10.1126/sciadv.abi9119
- Nothdurfter D, Ploner C, Coraça-Huber DC, et al. 3D bioprinted, vascularized neuroblastoma tumor environment in fluidic chip devices for precision medicine drug testing. Biofabrication. 2022;14(3):035002. doi: 10.1088/1758-5090/ac5fb7
- Appelt-Menzel A, Cubukova A, Günther K, et al. Establishment of a human blood‒brain barrier coculture model mimicking the neurovascular unit using induced pluri- and multipotent stem cells. Stem Cell Rep. 2017;8(4):894-906. doi: 10.1016/j.stemcr.2017.02.021
- Cucullo L, Couraud PO, Weksler B, et al. Immortalized human brain endothelial cells and flow-based vascular modeling: a marriage of convenience for rational neurovascular studies. J Cereb Blood Flow Metab. 2008;28:312-328. doi: 10.1038/sj.jcbfm.9600525
- Tabet A, Mommer S, Vigil JA, Hallou C, Bulstrode H, Scherman OA. Mechanical characterization of human brain tissue and soft dynamic gels exhibiting electromechanical neuro-mimicry. Adv Healthc Mater. 2019;8(10):e1900068. doi: 10.1002/adhm.201900068
- Fallenstein GT, Hulce VD, Melvin JW. Dynamic mechanical properties of human brain tissue. J Biomech. 1969;2(3): 217-226. doi: 10.1016/0021-9290(69)90079-7
- Dzikowski L, Mirzaei R, Sarkar S, et al. Fibrinogen in the glioblastoma microenvironment contributes to the invasiveness of brain tumor-initiating cells. Brain Pathol. 2021;31(5):e12947. doi: 10.1111/bpa.12947
- Luckenbill-Edds L. Laminin and the mechanism of neuronal outgrowth. Brain Res Brain Res Rev. 1997;23(1-2):1-27. doi: 10.1016/S0165-0173(96)00013-6
- Le LV, Mkrtschjan MA, Russell B, Desai TA. Hang on tight: reprogramming the cell with microstructural cues. Biomed Microdevices. 2019;21(2):43. doi: 10.1007/s10544-019-0394-9
- Pizzo AM, Kokini K, Vaughn LC, Waisner BZ, Voytik- Harbin SL. Extracellular matrix (ECM) microstructural composition regulates local cell-ECM biomechanics and fundamental fibroblast behavior: a multidimensional perspective. J Appl Physiol. 2005;98(5):1909-1921. doi: 10.1152/japplphysiol.01137.2004
- Roeder BA. The Influence of Extracellular Matrix (ECM) Microstructure on the Macro and Microlevel Biomechanical Behavior of Tissue Constructs and Cell-ECM Interactions. Dissertation. Purdue University; 2003.
- Mokkapati S, Fleger-Weckmann A, Bechtel M, et al. Basement membrane deposition of nidogen 1 but not nidogen 2 requires the nidogen binding module of the laminin gamma1 chain. J Biol Chem. 2011;286(3):1911- 1918. doi: 10.1074/jbc.M110.149864
- Yurchenco PD, Patton BL. Developmental and pathogenic mechanisms of basement membrane assembly. Curr Pharm Des. 2009;15(12):1277-1294. doi: 10.2174/138161209787846766
- Wang LB, Karpova A, Gritsenko MA, et al. Proteogenomic and metabolomic characterization of human glioblastoma. Cancer Cell. 2021;39(4):509-528.e20. doi: 10.1016/j.ccell.2021.01.006
- Wu JI, Wang LH. Emerging roles of gap junction proteins connexins in cancer metastasis, chemoresistance and clinical application. J Biomed Sci. 2019;26:8. doi: 10.1186/s12929-019-0497-x
- Yunker CK, Golembieski W, Lemke N, et al. SPARC-induced increase in glioma matrix and decrease in vascularity are associated with reduced VEGF expression and secretion. Int J Cancer. 2008;122(12):2735-2743. doi: 10.1002/ijc.23450
- Arnold SA, Brekken RA. SPARC: a matricellular regulator of tumorigenesis. J Cell Commun Signal. 2009;3(3-4):255-273. doi: 10.1007/s12079-009-0072-4
- Sun Y, Li Q, Liu W, Zhang B. Relationship between fibrinogen level and its regulatory gene with Alzheimer’s disease and vascular dementia. J Int Med Res. 2020;48(2):211476875. doi: 10.1177/0300060520902578
- Golanov EV, Sharpe MA, Regnier-Golanov AS, Del Zoppo GJ, Baskin DS, Britz GW. Fibrinogen chains intrinsic to the brain. Front Neurosci. 2019;13:541. doi: 10.3389/fnins.2019.00541
- Xie Y, He L, Lugano R, et al. Key molecular alterations in endothelial cells in human glioblastoma uncovered through single-cell RNA sequencing. JCI Insight. 2021;6(15):e150861. doi: 10.1172/jci.insight.150861
- Tanase C, Enciu AM, Codrici E, et al. Fatty acids, CD36, thrombospondin-1, and CD47 in glioblastoma: together and/or separately? Int J Mol Sci. 2022;23(2):604. doi: 10.3390/ijms23020604
- Shergalis A, Bankhead A, Luesakul U, Muangsin N, Neamati N. Current challenges and opportunities in treating glioblastoma. Pharmacol Rev. 2018;70(3):412-445. doi: 10.1124/pr.117.014944
- Abudumijiti A, Jianhua Rn, Yuwei Y, et al. Ganoderic acid improves 5-fluorouracil-induced cognitive dysfunction in mice. Food Funct. 2021;12:12325-12337. doi: 10.1039/D1FO03055H
- Cai YY, Yap CW, Wang Z, et al. Solubilization of vorinostat by cyclodextrins. J Clin Pharm Ther. 2010;35(5):521-526. doi: 10.1111/j.1365-2710.2009.01095.x
- Rompicharla SVK, Trivedi P, Kumari P, et al. Evaluation of anti-tumor efficacy of vorinostat encapsulated self-assembled polymeric micelles in solid tumors. AAPS PharmSciTech. 2018;19(7):3141-3151. doi: 10.1208/s12249-018-1149-2
- Wefel JS, Saleeba AK, Buzdar AU, Meyers CA. Acute and late onset cognitive dysfunction associated with chemotherapy in women with breast cancer. Cancer. 2010;116(14):3348- 3356. doi: 10.1002/cncr.25098
- Schagen SB, Muller MJ, Boogerd W, Mellenbergh GJ, van Dam FSAM. Change in cognitive function after chemotherapy: a prospective longitudinal study in breast cancer patients. J Natl Cancer Inst. 2006;98(23):1742-1745. doi: 10.1093/jnci/djj470
- Collins B, MacKenzie J, Tasca GA, Scherling C, Smith A. Cognitive effects of chemotherapy in breast cancer patients: a dose‒response study. Psychooncology. 2013;22(7):1517-1527. doi: 10.1002/pon.3163
- Wang H, Liu Z, Li A, et al. COL4A1 as a novel oncogene associated with the clinical characteristics of malignancy predicts poor prognosis in glioma. Exp Ther Med. 2021;22(5):1224. doi: 10.3892/etm.2021.10658
- Baker SJ, Poulikakos PI, Irie HY, Parekh S, Reddy EP. CDK4: a master regulator of the cell cycle and its role in cancer. Genes Cancer. 2022;25(13):21-45. doi: 10.18632/genesandcancer.221
- Fan Y, Peng X, Wang Y, Li B, Zhao G. Comprehensive analysis of HDAC family identifies HDAC1 as a prognostic and immune infiltration indicator and HDAC1-related signature for prognosis in glioma. Front Mol Biosci. 2021;8:720020. doi: 10.3389/fmolb.2021.720020
- Hrzenjak A, Moinfar F, Kremser ML, et al. Histone deacetylase inhibitor vorinostat suppresses the growth of uterine sarcomas in vitro and in vivo. Mol Cancer. 2010;9:49. doi: 10.1186/1476-4598-9-49
- Xu WS, Parmigiani R, Marks P. Histone deacetylase inhibitors: molecular mechanisms of action. Oncogene. 2007;26:5541-5552. doi: 10.1038/sj.onc.1210620
- Yin D, Ong JM, Hu J, et al. Suberoylanilide hydroxamic acid, a histone deacetylase inhibitor: effects on gene expression and growth of glioma cells in vitro and in vivo. Clin Cancer Res. 2007;13(3):1045-1052. doi: 10.1158/1078-0432.CCR-06-1261
- Kang DW, Hwang WC, Noh YN, et al. Phospholipase D1 is upregulated by vorinostat and confers resistance to vorinostat in glioblastoma. J Cell Physiol. 2021; 236(1):549-560. doi: 10.1002/jcp.29882
- Huang CY, Huang CY, Pai YC, et al. Glucose metabolites exert opposing roles in tumor chemoresistance. Front Oncol. 2019;9:1282. doi: 10.3389/fonc.2019.01282
- Miura K, Kinouchi M, Ishida K, et al. 5-FU metabolism in cancer and orally administrable 5-FU drugs. Cancers. 2010;2:1717-1730. doi: 10.3390/cancers2031717