Effect of in situ electromagnetic field manipulation on the microstructure and hardness of titanium alloy during laser melting deposition
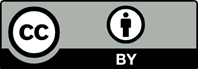
The electromagnetic field is a non-contact physical field that can influence the internal flow of the melt pool and regulate the microstructure properties of alloy through electromagnetic force during laser melting deposition (LMD). This study proposes a 3D numerical model of LMD Ti-6Al-4V coupled with an electromagnetic field and investigates the effect of the electromagnetic field on the fluid dynamics of the melt pool during LMD. The results indicated that a steady electromagnetic field can suppress the internal flow of the melt pool. In an electromagnetic field of 39.40 mT, the length of β-columnar grains significantly decreases from 490 to 354 μm, resulting in fragmentation and equiaxed tendencies, thereby enhancing the hardness of the deposition layer. This study provides a new method for in situ manipulation of the microstructure and mechanical properties of titanium alloys during LMD.
- Tan C, Li R, Su J, et al. Review on field assisted metal additive manufacturing. Int J Mach Tools Manuf. 2023;189:104032. doi: 10.1016/j.ijmachtools.2023.104032
- Zhang M, Wang B, Li X, Jiao G, Fang X, Huang H. Grain refinement of NiTi alloys during ultrasound-assisted wire-arc directed energy deposition. Virtual Phys Prototyp. 2024;19(1):e2289465. doi: 10.1080/17452759.2023.2289465
- Santos EC, Shiomi M, Osakada K, Laoui T. Rapid manufacturing of metal components by laser forming. Int J Mach Tools Manuf. 2006;46(12):1459-1468. doi: 10.1016/j.ijmachtools.2005.09.005
- Thompson SM, Bian L, Shamsaei N, Yadollahi A. An overview of direct laser deposition for additive manufacturing; Part I: Transport phenomena, modeling and diagnostics. Addit Manuf. 2015;8:36-62. doi: 10.1016/j.addma.2015.07.001
- Wang G, Zhao H, Liang H, Deng C, Ma W. Multi-objective optimisation of process parameters for laser-based directed energy deposition of a mixture of H13 and M2 steel powders on 4Cr5Mo2SiV1 steel. Virtual Phys Prototyp. 2024;19(1):e2290184. doi: 10.1080/17452759.2023.2290184
- Su J, Li Q, Teng J, et al. Programmable mechanical properties of additively manufactured novel steel. Int J Extrem Manuf. 2024;7(1):015001. doi: 10.1088/2631-7990/ad88bc
- Haley J, Karandikar J, Herberger C, MacDonald E, Feldhausen T, Lee Y. Review of in situ process monitoring for metal hybrid directed energy deposition. J Manuf Processes. 2024;109:128-139. doi: 10.1016/j.jmapro.2023.12.004
- Azarniya A, Colera XG, Mirzaali MJ, et al. Additive manufacturing of Ti-6Al-4V parts through laser metal deposition (LMD): Process, microstructure, and mechanical properties. J Alloys Compounds. 2019;804:163-191. doi: 10.1016/j.jallcom.2019.04.255
- Choi YR, Sun SD, Liu Q, Brandt M, Qian M. Influence of deposition strategy on the microstructure and fatigue properties of laser metal deposited Ti-6Al-4V powder on Ti-6Al-4V substrate. Int J Fatigue. 2020;130:105236. doi: 10.1016/j.ijfatigue.2019.105236
- Zhou X, Xu D, Geng S, et al. Mechanical properties, corrosion behavior and cytotoxicity of Ti-6Al-4V alloy fabricated by laser metal deposition. Mater Characterization. 2021;179:111302. doi: 10.1016/j.matchar.2021.111302
- Dinda GP, Dasgupta AK, Mazumder J. Laser aided direct metal deposition of Inconel 625 superalloy: Microstructural evolution and thermal stability. Mater Sci Eng A. 2009;509(1):98-104. doi: 10.1016/j.msea.2009.01.009
- Rao J, Leong Sing S, Liu P, Wang J, Sohn H. Non-destructive testing of metal-based additively manufactured parts and processes: A review. Virtual Phys Prototyp. 2023;18(1):e2266658. doi: 10.1080/17452759.2023.2266658
- Chen C, Sun H, Zhang Z, Zhao Y, Liu Y, Chen H. Grain structure control of TC11 alloy in laser direct energy deposition by a static magnetic field. Materialia. 2024;38:102267. doi: 10.1016/j.mtla.2024.102267
- Chen C, Zhang K, Zhao R, et al. Laser powder bed fusion of GH4099 Ni-based superalloy under a static magnetic field with tailored microstructure and enhanced mechanical performance. Virtual Phys Prototyp. 2024;19(1):e2411023. doi: 10.1080/17452759.2024.2411023
- Qin Y, Liao Y, Li G, Cui J, Jiang H. Numerical simulation and parameter analysis of electromagnetic riveting process for Ti-6Al-4V titanium rivet. Coatings. 2021;11(8):878. doi: 10.3390/coatings11080878
- Li Y, Xu W, Su Q, Wang Q. Microstructure and properties of Ti-Al coating on titanium alloy surface assisted by electromagnetic field. ACS Omega. 2024;9(46):46176-46191. doi: 10.1021/acsomega.4c06757
- Song X, Qi H, Li S, Hu Y, Yang W, Li Z. Effect of cryogenic coupled magnetic field treatment on the microstructure and mechanical properties on Ti-6Al-4V titanium alloy. Mater Today Commun. 2024;40:109417. doi: 10.1016/j.mtcomm.2024.109417
- Bachmann M, Avilov V, Gumenyuk A, Rethmeier M. Numerical assessment and experimental verification of the influence of the Hartmann effect in laser beam welding processes by steady magnetic fields. Int J Therm Sci. 2016;101:24-34. doi: 10.1016/j.ijthermalsci.2015.10.030
- Velde O, Gritzki R, Grundmann R. Numerical investigations of Lorentz force influenced Marangoni convection relevant to aluminum surface alloying. Int J Heat Mass Transfer. 2001;44(14):2751-2762.doi: 10.1016/S0017-9310(00)00299-4
- Kelly SM, Kampe SL. Microstructural evolution in laser-deposited multilayer Ti-6Al-4V builds: Part II. Thermal modeling. Metall Mater Transact A. 2004;35(6):1869-1879. doi: 10.1007/s11661-004-0094-8
- Wirth F, Wegener K. A physical modeling and predictive simulation of the laser cladding process. Addit Manuf. 2018;22:307-319. doi: 10.1016/j.addma.2018.05.017
- Hirt CW, Nichols BD. Volume of fluid (VOF) method for the dynamics of free boundaries. J Comput Phys. 1981;39(1):201-225. doi: 10.1016/0021-9991(81)90145-5
- Pinkerton AJ. Advances in the modeling of laser direct metal deposition. J Laser Appl. 2014;27(S1):S15001. doi: 10.2351/1.4815992
- Sun Z, Guo W, Li L. Numerical modelling of heat transfer, mass transport and microstructure formation in a high deposition rate laser directed energy deposition process. Addit Manuf. 2020;33:101175. doi: 10.1016/j.addma.2020.101175
- Wei S, Wang G, Shin YC, Rong Y. Comprehensive modeling of transport phenomena in laser hot-wire deposition process. Int J Heat Mass Transfer. 2018;125:1356-1368. doi: 10.1016/j.ijheatmasstransfer.2018.04.164
- Morville S, Carin M, Peyre P, et al. 2D longitudinal modeling of heat transfer and fluid flow during multilayered direct laser metal deposition process. J Laser Appl. 2012;24:032008. doi: 10.2351/1.4726445
- Gan Z, Liu H, Li S, He X, Yu G. Modeling of thermal behavior and mass transport in multi-layer laser additive manufacturing of Ni-based alloy on cast iron. Int J Heat Mass Transfer. 2017;111:709-722. doi: 10.1016/j.ijheatmasstransfer.2017.04.055
- Gan Z, Yu G, He X, Li S. Surface-active element transport and its effect on liquid metal flow in laser-assisted additive manufacturing. Int Commun Heat Mass Transfer. 2017;86:206-214. doi: 10.1016/j.icheatmasstransfer.2017.06.007
- Arrizubieta JI, Lamikiz A, Klocke F, Martínez S, Arntz K, Ukar E. Evaluation of the relevance of melt pool dynamics in laser material deposition process modeling. Int J Heat Mass Transfer. 2017;115:80-91. doi: 10.1016/j.ijheatmasstransfer.2017.07.011
- Bai X, Colegrove P, Ding J, et al. Numerical analysis of heat transfer and fluid flow in multilayer deposition of PAW-based wire and arc additive manufacturing. Int J Heat Mass Transfer. 2018;124:504-516. doi: 10.1016/j.ijheatmasstransfer.2018.03.085
- Saldi ZS. Marangoni Driven Free Surface Flows in Liquid Weld Pools. The Netherlands: Delft University of Technology; 2012. Available from: https://resolver.tudelft. nl/uuid:8401374b-9e9c-4d25-86b7-fc445ec73d27
- Spiegel EA, Veronis G. On the boussinesq approximation for a compressible fluid. Astrophys J. 1960;131:442. doi: 10.1086/146849
- Boivineau M, Cagran C, Doytier D, et al. Thermophysical properties of solid and liquid Ti-6Al-4V (TA6V) alloy. Int J Thermophys. 2006;27(2):507-529. doi: 10.1007/PL00021868
- Hagqvist P, Sikström F, Christiansson AK. Emissivity estimation for high temperature radiation pyrometry on Ti-6Al-4V. Measurement. 2013;46(2):871-880. doi: 10.1016/j.measurement.2012.10.019
- Soylemez E, Beuth J, Taminger K. Controlling Melt Pool Dimensions Over a Wide Range of Material Deposition Rates in Electron Beam Additive Manufacturing. In: International Solid Freeform Fabrication Symposium; 2010. doi: 10.26153/tsw/15221
- Tang Q, Pang S, Chen B, Suo H, Zhou J. A three dimensional transient model for heat transfer and fluid flow of weld pool during electron beam freeform fabrication of Ti-6-Al-4-V alloy. Int J Heat Mass Transfer. 2014;78:203-215. doi: 10.1016/j.ijheatmasstransfer.2014.06.048
- Liu C, Xie L, Qian D, et al. Microstructure evolution and mechanical property response of TC11 titanium alloy under electroshock treatment. Mater Design. 2021;198:109322. doi: 10.1016/j.matdes.2020.109322
- Wen Y, Sun X, Zhou J, et al. Influence of electroshocking treatment on microstructure and mechanical properties of Ti-6.5 Al-3.5 Mo-1.5 Zr-0.3 si thin-wall specimen manufactured by laser melting deposition. Acta Metall Sin (Engl Lett). 2024;37(1):145-158. doi: 10.1007/s40195-023-01592-x
- Liu C, Yin F, Xie L, et al. Evolution of grain boundary and texture in TC11 titanium alloy under electroshock treatment. J Alloys Compounds. 2022;904:163969. doi: 10.1016/j.jallcom.2022.163969
- Zhou J, Liu C, Wu Y, et al. Evolution mechanism of grain orientation and texture distribution of Ti-6.5 Al-3.5 Mo-1.5 Zr-0.3 Si alloy under electroshocking treatment. J Mater Res Technol. 2023;25:5693-5704. doi: 10.1016/j.jmrt.2023.07.028