3D-bioprinted in vitro skeletal muscle with pennate fiber architecture to enhance contractile function
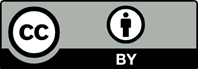
Correction for this article is available: view correction
Skeletal muscle tissue engineering (SMTE) has important research value and broad applicational prospects in areas such as muscle repair, disease modeling, drug testing, and biohybrid robotics. Despite advances in research on engineered skeletal muscles, it remains challenging to improve their functional performance, especially relatively large-sized muscles. Inspired by pennate muscles with a large force output capacity, a novel in vitro skeletal muscle tissue design mimicking the macro and microstructures of the gastrocnemius muscle in frogs was proposed and optimized through simulation. The cell-laden hydrogel was then 3D-bioprinted to fabricate tissues with fusiform geometry and induced microchannels with a pennate angle of 15°. The morphology, cell status, and contraction performance of 3D-bioprinted muscle tissues were evaluated after electrical stimulation, which induced the directional alignment of myotubes. The results indicated that our 3D-bioprinted pennate skeletal muscle tissues exhibited high cell viability (79.89%) and alignment of muscle fibers (51.93%), with a maximum contraction force of 443.085 μN, almost twice the force of 3D-printed parallel muscle tissues in our study. This work will support the exploration of design strategies and rapid manufacturing techniques for next-generation SMTEs with enhanced functional performance.

- Samandari M, Quint J, Rodríguez-delaRosa A, Sinha I, Pourquié O, Tamayol A. Bioinks and bioprinting strategies for skeletal muscle tissue engineering. Adv Mater. 2022;34(12):2105883. doi: 10.1002/adma.202105883
- Derakhshanfar S, Mbeleck R, Xu K, Zhang X, Zhong W, Xing M. 3D bioprinting for biomedical devices and tissue engineering: a review of recent trends and advances. Bioact Mater. 2018;3(2):144-156. doi: 10.1016/j.bioactmat.2017.11.008
- Corona BT, Rivera JC, Owens JG, Wenke JC, Rathbone CR. Volumetric muscle loss leads to permanent disability following extremity trauma. J Rehabil Res Dev. 2015;52(7):785-792. doi: 10.1682/jrrd.2014.07.0165
- Liu J, Saul D, Böker KO, Ernst J, Lehman W, Schilling AF. Current methods for skeletal muscle tissue repair and regeneration. Biomed Res Int. 2018;11:1984879. doi: 10.1155/2018/1984879
- Zhuang P, An J, Chua CK, Tan LP. Bioprinting of 3D in vitro skeletal muscle models: a review. Mater Des. 2020;193:108794. doi: 10.1016/j.matdes.2020.108794
- Kwee BJ, Mooney DJ. Biomaterials for skeletal muscle tissue engineering. Curr Opin Biotechnol. 2017;47:16-22. doi: 10.1016/j.copbio.2017.05.003
- Gholobova D, Terrie L, Gerard M, Declercq H, Thorrez L. Vascularization of tissue-engineered skeletal muscle constructs. Biomaterials. 2020;235:119708. doi: 10.1016/j.biomaterials.2019.119708
- Kang MS, Yu Y, Park R, et al. Highly aligned ternary nanofiber matrices loaded with MXene expedite regeneration of volumetric muscle loss. Nanomicro Lett. 2024;16(1):73. doi: 10.1007/s40820-023-01293-1
- Ricotti L, Trimmer B, Feinberg AW, et al. Biohybrid actuators for robotics: a review of devices actuated by living cells. Sci Robot. 2017;2(12):eaaq0495. doi: 10.1126/scirobotics.aaq0495
- Ostrovidov S, Hosseini V, Ahadian S, et al. Skeletal muscle tissue engineering: methods to form skeletal myotubes and their applications. Tissue Eng Part B Rev. 2014;20(5):403-436. doi: 10.1089/ten.teb.2013.0534
- Rao LJ, Qian Y, Khodabukus A, Ribar T, Bursac N. Engineering human pluripotent stem cells into a functional skeletal muscle tissue. Nat Commun. 2018;9126. doi: 10.1038/s41467-017-02636-4
- Martin NRW, Turner MC, Farrington R, Player DJ, Lewis MP. Leucine elicits myotube hypertrophy and enhances maximal contractile force in tissue engineered skeletal muscle in vitro. J Cell Physiol. 2017;232(10):2788-2797. doi: 10.1002/jcp.25960
- Madden L, Juhas M, Kraus WE, Truskey GA, Bursac N. Bioengineered human myobundles mimic clinical responses of skeletal muscle to drugs. Elife. 2015;4:e04885. doi: 10.7554/eLife.04885
- Nagamine K, Kawashima T, Sekine S, Ido Y, Kanzaki M, Nishizawa M. Spatiotemporally controlled contraction of micropatterned skeletal muscle cells on a hydrogel sheet. Lab Chip. 2011;11(3):513-517. doi: 10.1039/c0lc00364f
- Kamm RD, Bashir R. Creating living cellular machines. Ann of Biome Eng. 2013;42(2):445-459. doi: 10.1007/s10439-013-0902-7
- Chan V, Asada HH, Bashir R. Utilization and control of bioactuators across multiple length scales. Lab Chip. 2014;14(4):653-670. doi: 10.1039/c3lc50989c
- Ricotti L, Menciassi A. Bio-hybrid muscle cell-based actuators. Biomed Microdevices. 2012;14(6):987-998. doi: 10.1007/s10544-012-9697-9
- Zupan M, Ashby MF, Fleck NA. Actuator classification and selection—the development of a database. Adv Eng Mater. 2002;4(12):933-940. doi: 10.1002/adem.200290009
- Coyle S, Majidi C, LeDuc P, Hsia KJ. Bio-inspired soft robotics: material selection, actuation, and design. Extreme Mech Lett. 2018;22:51-59. doi: 10.1016/j.eml.2018.05.003
- Kim Y, Yang Y, Zhang X, et al. Remote control of muscle-driven miniature robots with battery-free wireless optoelectronics. Sci Robot. 2023;8(74):eadd1053. doi: 10.1126/scirobotics.add1053
- Morimoto Y, Onoe H, Takeuchi S. Biohybrid robot powered by an antagonistic pair of skeletal muscle tissues. Sci Robot. 2018;3(18):eaat4440. doi: 10.1126/scirobotics.aat4440
- Gao GF, Cui XF. Three-dimensional bioprinting in tissue engineering and regenerative medicine. Biotechnol Lett. 2016;38(2):203-211. doi: 10.1007/s10529-015-1975-1
- Kim JH, Seol YJ, Ko IK, et al. 3D bioprinted human skeletal muscle constructs for muscle function restoration. Sci Rep. 2018;8(1):12307. doi: 10.1038/s41598-018-29968-5
- Kang HW, Lee SJ, Ko IK, Kengla C, Yoo JJ, Atala A. A 3D bioprinting system to produce human-scale tissue constructs with structural integrity. Nat Biotechnol. 2016;34(3):312-319. doi: 10.1038/nbt.3413
- Kim JH, Kim I, Seol YJ, et al. Neural cell integration into 3D bioprinted skeletal muscle constructs accelerates restoration of muscle function. Nat Commun. 2020;11(1)1025. doi: 10.1038/s41467-020-14930-9
- Bian WN, Liau B, Badie N, Bursac N. Mesoscopic hydrogel molding to control the 3D geometry of bioartificial muscle tissues. Nat Protoc. 2009;4(10):1522-1534. doi: 10.1038/nprot.2009.155
- Tanaka Y, Noguchi Y, Yalikun Y, Kamamichi N. Earthworm muscle driven bio-micropump. Sens Actuator B Chem. 2017;242:1186-1192. doi: 10.1016/j.snb.2016.09.123
- Liu L, Zhang C, Wang W, Xi N, Wang Y. Regulation of C2C12 differentiation and control of the beating dynamics of contractile cells for a muscle-driven biosyncretic crawler by electrical stimulation. Soft Robot. 2018;5(6):748-760. doi: 10.1089/soro.2018.0017
- Mita H, Mizuno Y, Tanaka H, Fujie T. UV laser-processed microstructure for building biohybrid actuators with anisotropic movement. Biofabrication. 2024;16(2):025010. doi: 10.1088/1758-5090/ad2080
- Roberts TJ, Eng CM, Sleboda DA, et al. The multi-scale, three-dimensional nature of skeletal muscle contraction. Physiology (Bethesda). 2019;34(6):402-408. doi: 10.1152/physiol.00023.2019
- Mestre R, Patiño T, Barceló X, Anand S, Pérez‐Jiménez A, Sánchez S. Force modulation and adaptability of 3d‐bioprinted biological actuators based on skeletal muscle tissue. Adv Mater Technol. 2018;4(2):1800631. doi: 10.1002/admt.201800631
- Markstedt K, Mantas A, Tournier I, Martínez Ávila H, Hägg D, Gatenholm P. 3D bioprinting human chondrocytes with nanocellulose–alginate bioink for cartilage tissue engineering applications. Biomacromolecules. 2015; 16(5):1489-1496. doi: 10.1021/acs.biomac.5b00188
- Kolesky DB, Truby RL, Gladman AS, Busbee TA, Homan KA, Lewis JA. 3D bioprinting of vascularized, heterogeneous cell‐laden tissue constructs. Adv Mater. 2014; 26(19):3124-3130. doi: 10.1002/adma.201305506
- Cernencu AI, Lungu A, Dragusin DM, et al. 3D bioprinting of biosynthetic nanocellulose-filled gelma inks highly reliable for soft tissue-oriented constructs. Materials. 2021;14(17):4891. doi: 10.3390/ma14174891
- Mao M, He J, Li Z, Han K, Li D. Multi-directional cellular alignment in 3D guided by electrohydrodynamically-printed microlattices. Acta Biomater. 2020;101:141-151. doi: 10.1016/j.actbio.2019.10.028
- Raman R, Cvetkovic C, Uzel SGM, et al. Optogenetic skeletal muscle-powered adaptive biological machines. Proc Natl Acad Sci. 2016;113(13):3497-3502. doi: 10.1073/pnas.1516139113
- Mestre R, Fuentes J, Lefaix L, et al. Improved performance of biohybrid muscle‐based bio‐bots doped with piezoelectric boron nitride nanotubes. Adv Mater Technol. 2022;8(2):2200505. doi: 10.1002/admt.202200505
- Cvetkovic C, Raman R, Chan V, et al. Three-dimensionally printed biological machines powered by skeletal muscle. Proc Natl Acad Sci U S A. 2014;111(28):10125-10130. doi: 10.1073/pnas.1401577111
- Pagan‐Diaz GJ, Zhang X, Grant L, et al. Simulation and fabrication of stronger, larger, and faster walking biohybrid machines. Adv Funct Mater. 2018;28(23):1801145. doi: 10.1002/adfm.201801145
- Wang J, Wang Y, Kim Y, Yu T, Bashir R. Multi-actuator light-controlled biological robots. APL Bioeng. 2022;6(3):036103. doi: 10.1063/5.0091507
- Choi YJ, Kim TG, Jeong J, et al. 3D cell printing of functional skeletal muscle constructs using skeletal muscle-derived bioink. Adv Healthc Mater. 2016;5(20):2636-2645. doi: 10.1002/adhm.201600483