Spatiotemporal delivery of BMP-2 and FGF-18 in 3D-bioprinted tri-phasic osteochondral scaffolds enhanced compartmentalized osteogenic and chondrogenic differentiation of mesenchymal stem cells isolated from rats with varied organizational morphologies
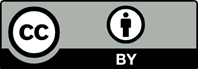
Replicating the heterogeneous structure and promoting compartmentalized osteogenesis/chondrogenesis are critical considerations in designing scaffolds for osteochondral tissue regeneration. However, desirable osteochondral regeneration cannot be achieved mainly due to the absence of effective delivery strategies for growth factors (GFs) and the insufficiency of desirable organizational morphologies for seed cells. Herein, we developed a tri-phasic osteochondral scaffold consisting of bone morphogenetic protein-2 (BMP-2)-loaded subchondral layer, fibroblast growth factor-18 (FGF-18)-loaded cartilage layer, and an interface layer that acted as a barrier to reduce the mutual interference of GFs, via cryogenic 3D bioprinting. BMP-2 could exert osteogenic effects for 14 days, and FGF-18 could exert chondrogenic effects for 21 days, demonstrating the time-controlled release function of BMP-2 and FGF-18. By further seeding discrete rat bone marrow mesenchymal stem cells (rBMSCs) and rBMSC microspheres, respectively, onto the subchondral layer and cartilage layer, the engineered cell-laden osteochondral tissue was constructed. The spatiotemporal release of BMP-2 and FGF-18 in the subchondral layer and cartilage layer promoted the osteogenic differentiation of discrete rBMSCs and chondrogenic differentiation of rBMSC microspheres in the subchondral layer and cartilage layer, respectively. In summary, by seeding rBMSCs with varied organizational morphologies in 3D-printed osteochondral scaffolds with a spatiotemporally controlled strategy, engineered osteochondral tissue with compartmentalized osteogenic/chondrogenic differentiation potent can be formed, displaying a facile and promising way to achieve desirable osteochondral tissue regeneration.
- Olstad K, Ekman S, Carlson CS. An update on the pathogenesis of osteochondrosis. Vet Pathol. 2015;52(5):7 85-802. doi: 10.1177/0300985815588778
- He Y, Li Z, Alexander PG, et al. Pathogenesis of osteoarthritis: risk factors, regulatory pathways in chondrocytes, and experimental models. Biology. 2020;9(8):194. doi: 10.3390/biology9080194
- Abramoff B, Caldera FE. Osteoarthritis pathology, diagnosis, and treatment options. Méd Clin North Am. 2020;104(2):293-311. doi: 10.1016/j.mcna.2019.10.007
- Hermann W, Lambova S, Muller-Ladner U. Current treatment options for osteoarthritis. Curr Rheumatol Rev. 2017;14(2):108-116. doi: 10.2174/1573397113666170829155149
- Madry H. Surgical therapy in osteoarthritis. Osteoarthr Cartil. 2022;30(8):1019-1034. doi: 10.1016/j.joca.2022.01.012
- Wei W, Dai H. Articular cartilage and osteochondral tissue engineering techniques: recent advances and challenges. Bioact Mater. 2021;6(12):4830-4855. doi: 10.1016/j.bioactmat.2021.05.011
- Zhou L, Gjvm VO, Malda J, et al. Innovative tissue‐engineered strategies for osteochondral defect repair and regeneration: current progress and challenges. Adv Healthc Mater. 2020;9(23):e2001008. doi: 10.1002/adhm.202001008
- Colella F, Garcia JP, Sorbona M, et al. Drug delivery in intervertebral disc degeneration and osteoarthritis: selecting the optimal platform for the delivery of disease-modifying agents. J Control Release. 2020;328:985-999. doi: 10.1016/j.jconrel.2020.08.041
- Rahimi M, Charmi G, Matyjaszewski K, Banquy X, Pietrasik J. Recent developments in natural and synthetic polymeric drug delivery systems used for the treatment of osteoarthritis. Acta Biomater. 2021;123:31-50. doi: 10.1016/j.actbio.2021.01.003
- Zhan A, Chen L, Sun W, et al. Enhancement of diabetic wound healing using a core-shell nanofiber platform with sequential antibacterial, angiogenic, and collagen deposition activities. Mater Des. 2022;218:110660. doi: 10.1016/j.matdes.2022.110660
- He W, Li C, Zhao S, et al. Integrating coaxial electrospinning and 3D printing technologies for the development of biphasic porous scaffolds enabling spatiotemporal control in tumor ablation and osteochondral regeneration. Bioact Mater. 2024;34:338-353. doi: 10.1016/j.bioactmat.2023.12.020
- Li S, Zheng W, Deng W, et al. Logic‐based strategy for spatiotemporal release of dual extracellular vesicles in osteoarthritis treatment. Adv Sci. (Weinh). 2024:e2403227. doi: 10.1002/advs.202403227
- Bhat BB, Mehta CH, Suresh A, Velagacherla V, Nayak UY. Controlled release technologies for chronotherapy: current status and future perspectives. Curr Pharm Des. 2023;29(14):1069-1091.doi: 10.2174/1381612829666230423144232
- Santo VE, Gomes ME, Mano JF, Reis RL. From nano- to macro-scale: nanotechnology approaches for spatially controlled delivery of bioactive factors for bone and cartilage engineering. Nanomedicine. 2012;7(7):1045-1066. doi: 10.2217/nnm.12.78
- Lu S, Lam J, Trachtenberg JE, et al. Dual growth factor delivery from bilayered, biodegradable hydrogel composites for spatially-guided osteochondral tissue repair. Biomaterials. 2014;35(31):8829-8839. doi: 10.1016/j.biomaterials.2014.07.006
- Fortier LA, Barker JU, Strauss EJ, McCarrel TM, Cole BJ. The role of growth factors in cartilage repair. Clin Orthop Relat Res. 2011;469(10):2706-2715. doi: 10.1007/s11999-011-1857-3
- Devescovi V, Leonardi E, Ciapetti G, Cenni E. Growth factors in bone repair. Chir Organi Mov. 2008;92(3):161-168. doi: 10.1007/s12306-008-0064-1
- Kim S, Lee S, Kim K. Cutting-edge enabling technologies for regenerative medicine. Adv Exp Med Biol. 2018;1078: 233-244. doi: 10.1007/978-981-13-0950-2_12
- Augustyniak E, Trzeciak T, Richter M, Kaczmarczyk J, Suchorska W. The role of growth factors in stem cell-directed chondrogenesis: a real hope for damaged cartilage regeneration. Int Orthop. 2015;39(5):995-1003. doi: 10.1007/s00264-014-2619-0
- Ansari S, Khorshidi S, Karkhaneh A. Engineering of gradient osteochondral tissue: from nature to lab. Acta Biomater. 2019;87:41-54. doi: 10.1016/j.actbio.2019.01.071
- Hoemann C, Lafantaisie-Favreau CH, Lascau-Coman V, Chen G, Guzmán-Morales J. The cartilage-bone interface. J Knee Surg. 2012;25(2):85-98. doi: 10.1055/s-0032-1319782
- Seong JM, Kim BC, Park JH, Kwon IK, Mantalaris A, Hwang YS. Stem cells in bone tissue engineering. Biomed Mater. 2010;5(6):062001. doi: 10.1088/1748-6041/5/6/062001
- Li Q, Xu S, Feng Q, et al. 3D printed silk-gelatin hydrogel scaffold with different porous structure and cell seeding strategy for cartilage regeneration. Bioact Mater. 2021;6(10):3396-3410. doi: 10.1016/j.bioactmat.2021.03.013
- Sarem M, Otto O, Tanaka S, Shastri VP. Cell number in mesenchymal stem cell aggregates dictates cell stiffness and chondrogenesis. Stem Cell Res Ther. 2019;10(1):10. doi: 10.1186/s13287-018-1103-y
- Karim A, Amin AK, Hall AC. The clustering and morphology of chondrocytes in normal and mildly degenerate human femoral head cartilage studied by confocal laser scanning microscopy. J Anat. 2018;232(4):686-698.doi: 10.1111/joa.12768
- Xiang XN, Zhu SY, He HC, Yu X, Xu Y, He CQ. Mesenchymal stromal cell-based therapy for cartilage regeneration in knee osteoarthritis. Stem Cell Res Ther. 2022; 13(1):14. doi: 10.1186/s13287-021-02689-9
- Liu Y, Zhou G, Cao Y. Recent progress in cartilage tissue engineering—our experience and future directions. Engineering. 2017;3(1):28-35. doi: 10.1016/j.eng.2017.01.010
- Buchanan JL. Types of fibrocartilage. Clin Podiatr Med Surg. 2022;39(3):357-361. doi: 10.1016/j.cpm.2022.02.001
- Kheir E, Shaw D. Hyaline articular cartilage. Orthop Trauma. 2009;23(6):450-455. doi: 10.1016/j.mporth.2009.01.003
- Armiento AR, Alini M, Stoddart MJ. Articular fibrocartilage - why does hyaline cartilage fail to repair? Adv Drug Deliv Rev. 2019;146:289-305. doi: 10.1016/j.addr.2018.12.015
- Osch GJVMV, Brittberg M, Dennis JE, et al. Cartilage repair: past and future – lessons for regenerative medicine. J Cell Mol Med. 2009;13(5):792-810. doi: 10.1111/j.1582-4934.2009.00789.x
- Goodson HV, Jonasson EM. Microtubules and microtubule-associated proteins. Cold Spring Harb Perspect Biol. 2018;10(6):a022608. doi: 10.1101/cshperspect.a022608
- Li J, Jiang H, Lv Z, et al. Articular fibrocartilage-targeted therapy by microtubule stabilization. Sci Adv. 2022;8(46):eabn8420. doi: 10.1126/sciadv.abn8420
- Li J, Fan C, Lv Z, et al. Microtubule stabilization targeting regenerative chondrocyte cluster for cartilage regeneration. Theranostics. 2023;13(10):3480-3496. doi: 10.7150/thno.85077
- Hall AC. The role of chondrocyte morphology and volume in controlling phenotype—implications for osteoarthritis, cartilage repair, and cartilage engineering. Curr Rheumatol Rep. 2019;21(8):38. doi: 10.1007/s11926-019-0837-6
- Yang Y, Zheng W, Tan W, et al. Injectable MMP1-sensitive microspheres with spatiotemporally controlled exosome release promote neovascularized bone healing. Acta Biomater. 2023;157:321-336. doi: 10.1016/j.actbio.2022.11.065
- Beck EC, Barragan M, Tadros MH, Gehrke SH, Detamore MS. Approaching the compressive modulus of articular cartilage with a decellularized cartilage-based hydrogel. Acta Biomater. 2016;38: 94-105. doi: 10.1016/j.actbio.2016.04.019
- Little CJ, Bawolin NK, Chen X. Mechanical properties of natural cartilage and tissue-engineered constructs. Tissue Eng Part B: Rev. 2011;17(4):213-227. doi: 10.1089/ten.teb.2010.0572
- Keaveny TM, Hayes WC. A 20-year perspective on the mechanical properties of trabecular bone. J Biomech Eng. 1993;115(4B):534-542. doi: 10.1115/1.2895536
- Rezwan K, Chen QZ, Blaker JJ, Boccaccini AR. Biodegradable and bioactive porous polymer/inorganic composite scaffolds for bone tissue engineering. Biomaterials. 2006;27(18): 3413-3431. doi: 10.1016/j.biomaterials.2006.01.039
- Zhang N, Wang Y, Zhang J, Guo J, He J. Controlled domain gels with a biomimetic gradient environment for osteochondral tissue regeneration. Acta Biomater. 2021;135:304-317. doi: 10.1016/j.actbio.2021.08.029
- Yildirim N, Amanzhanova A, Kulzhanova G, Mukasheva F, Erisken C. Osteochondral interface: regenerative engineering and challenges. ACS Biomater Sci Eng. 2023;9(3):1205-1223. doi: 10.1021/acsbiomaterials.2c01321
- Niu X, Li N, Du Z, Li X. Integrated gradient tissue-engineered osteochondral scaffolds: Challenges, current efforts and future perspectives. Bioact Mater. 2023;20: 574-597. doi: 10.1016/j.bioactmat.2022.06.011
- Santos-Beato P, Midha S, Pitsillides AA, Miller A, Torii R, Kalaskar DM. Biofabrication of the osteochondral unit and its applications: current and future directions for 3D bioprinting. J Tissue Eng. 2022;13:20417314221133480. doi: 10.1177/20417314221133480
- Vyas C, Mishbak H, Cooper G, Peach C, Pereira RF, Bartolo P. Biological perspectives and current biofabrication strategies in osteochondral tissue engineering. Biomanufacturing Rev. 2020;5(1):2. doi: 10.1007/s40898-020-00008-y
- Wang C, Huang W, Zhou Y, et al. 3D printing of bone tissue engineering scaffolds. Bioact Mater. 2020;5(1):82-91. doi: 10.1016/j.bioactmat.2020.01.004
- Zaszczyńska A, Moczulska-Heljak M, Gradys A, Sajkiewicz P. Advances in 3D printing for tissue engineering. Materials (Basel). 2021;14(12):3149. doi: 10.3390/ma14123149
- Quan H, Zhang T, Xu H, Luo S, Nie J, Zhu X. Photo-curing 3D printing technique and its challenges. Bioact Mater. 2020;5(1):110-115. doi: 10.1016/j.bioactmat.2019.12.003
- Li Z, Xu M, Wang J, Zhang F. Recent advances in cryogenic 3D printing technologies. Adv Eng Mater. 2022;24(10):2200245. doi: 10.1002/adem.202200245
- Maia FR, Bastos AR, Oliveira JM, Correlo VM, Reis RL. Recent approaches towards bone tissue engineering. Bone. 2022;154:116256. doi: 10.1016/j.bone.2021.116256
- Wasyłeczko M, Sikorska W, Chwojnowski A. Review of synthetic and hybrid scaffolds in cartilage tissue engineering. Membranes (Basel). 2020;10(11):348. doi: 10.3390/membranes10110348
- Wang C, Yue H, Huang W, et al. Cryogenic 3D printing of heterogeneous scaffolds with gradient mechanical strengths and spatial delivery of osteogenic peptide/TGF- 1 for osteochondral tissue regeneration. Biofabrication. 2020;12(2):025030. doi: 10.1088/1758-5090/ab7ab5
- Li D, Guo Y, Lu H, et al. The effect of local delivery of adiponectin from biodegradable microsphere–scaffold composites on new bone formation in adiponectin knockout mice. J Mater Chem B. 2016;4(27):4771-4779. doi: 10.1039/c6tb00704j
- Yu X, Tang X, Gohil SV, Laurencin CT. Biomaterials for bone regenerative engineering. Adv Healthc Mater. 2015;4(9):1268-1285. doi: 10.1002/adhm.201400760
- Salaris V, Leonés A, Lopez D, Kenny JM, Peponi L. Shape-memory materials via electrospinning: a review. Polymers (Basel). 2022;14(5):995. doi: 10.3390/polym14050995
- Pérez-Luna VH, González-Reynoso O. Encapsulation of biological agents in hydrogels for therapeutic applications. Gels. 2018;4(3):61. doi: 10.3390/gels4030061
- Ryoo HM, Lee MH, Kim YJ. Critical molecular switches involved in BMP-2-induced osteogenic differentiation of mesenchymal cells. Gene. 2006;366(1):51-57. doi: 10.1016/j.gene.2005.10.011
- Wu M, Chen F, Liu H, et al. Bioinspired sandwich-like hybrid surface functionalized scaffold capable of regulating osteogenesis, angiogenesis, and osteoclastogenesis for robust bone regeneration. Mater Today Bio. 2022; 17:100458. doi: 10.1016/j.mtbio.2022.100458
- Lu Q, Diao J, Wang Y, et al. 3D printed pore morphology mediates bone marrow stem cell behaviors via RhoA/ROCK2 signaling pathway for accelerating bone regeneration. Bioact Mater. 2023;26:413-424. doi: 10.1016/j.bioactmat.2023.02.025
- Bradley EW, Carpio LR, Newton AC, Westendorf JJ. Deletion of the PH-domain and Leucine-rich repeat protein phosphatase 1 (Phlpp1) increases fibroblast growth factor (Fgf) 18 expression and promotes chondrocyte proliferation*. J Biol Chem. 2015;290(26):16272-16280. doi: 10.1074/jbc.m114.612937
- Davidson D, Blanc A, Filion D, et al. Fibroblast growth factor (FGF) 18 signals through FGF receptor 3 to promote chondrogenesis*. J Biol Chem. 2005;280(21): 20509-20515. doi: 10.1074/jbc.m410148200
- Einhorn TA, Gerstenfeld LC. Fracture healing: mechanisms and interventions. Nat Rev Rheumatol. 2015;11(1):45-54. doi: 10.1038/nrrheum.2014.164
- Li M, Yin H, Yan Z, et al. The immune microenvironment in cartilage injury and repair. Acta Biomater. 2022;140: 23-42. doi: 10.1016/j.actbio.2021.12.006
- Taskin MB, Klausen LH, Dong M, Chen M. Emerging wet electrohydrodynamic approaches for versatile bioactive 3D interfaces. Nano Res. 2020;13(2):315-327. doi: 10.1007/s12274-020-2635-x
- Lee S, Lee K, Kim SH, Jung Y. Enhanced cartilaginous tissue formation with a cell aggregate-fibrin-polymer scaffold complex. Polymers (Basel). 2017;9(8):348. doi: 10.3390/polym9080348
- Ghadially FN. Structure and function of articular cartilage. Clin Rheum Dis. 1981;7(1):3-28. doi: 10.1016/s0307-742x(21)00330-1
- Xiao S, Zhao T, Wang J, et al. Gelatin methacrylate (GelMA)- based hydrogels for cell transplantation: an effective strategy for tissue engineering. Stem Cell Rev Rep. 2019;15(5): 664-679. doi: 10.1007/s12015-019-09893-4
- Tibbitt MW, Anseth KS. Hydrogels as extracellular matrix mimics for 3D cell culture. Biotechnol Bioeng. 2009;103(4):655-663. doi: 10.1002/bit.22361