Horsetail-inspired lattice structures for bone scaffold applications
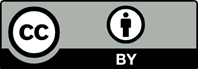
A well-design bone scaffold is critical for facilitating post in vivo implantation recovery. Key factors, such as elastic moduli matching to alleviate stress shielding, anisotropic characteristics, and sufficient porosity for cell ingrowth, shape the design consideration for bone scaffolds. Herein, we propose a novel body-centered cubic (BCC) lattice with modified horsetail inspired cross-section strut members as the building block for synthetic bone scaffold application. We demonstrated that geometrical parameters can be varied to attain expected desirable mechanical properties. We also successfully matched the performance of the physical compression tests of Ti-6Al-4V-based samples manufactured using selective laser melting to that of the simulation environment to facilitate design. Through our work, we created Ti-6Al-4V-based lattices, which match the mechanical performance of native bone in terms of elastic moduli and yield strength. Biologically, the lattices provide in-strut pore dimensions that facilitate bone cell ingrowth as well as yield point that is beyond the strain required to promote secondary healing. The good energy absorption capability of our lattices also adds resilience to accidental damage when applied for use in bone scaffold design. We also discovered that the isotropy characteristic is decoupled from the outer radius of the designed lattice; this avoids convolution that would otherwise increase design difficulties. Through this novel design, the tuning of the mechanical properties to attain the key considerations with geometrical variations is made possible.
- Corrado A, Cici D, Rotondo C, Maruotti N, Cantatore FP. Molecular basis of bone aging. Int J Mol Sci. 2020;21(10):3679. doi: 10.3390/ijms21103679
- Wu A-M, Bisignano C, James SL, et al. Global, regional, and national burden of bone fractures in 204 countries and territories, 1990–2019: a systematic analysis from the Global Burden of Disease Study 2019. Lancet Healthy Longev. 2021;2(9):e580-e592. doi: 10.1016/s2666-7568(21)00172-0
- Amini AR, Laurencin CT, Nukavarapu SP. Bone tissue engineering: recent advances and challenges. Crit Rev Biomed Eng. 2012;40(5):363-408. doi: 10.1615/critrevbiomedeng.v40.i5.10
- Dec P, Modrzejewski A, Pawlik A. Existing and novel biomaterials for bone tissue engineering. Int J Mol Sci. 2022;24(1):529. doi: 10.3390/ijms24010529
- Wang X, Xu S, Zhou S, et al. Topological design and additive manufacturing of porous metals for bone scaffolds and orthopaedic implants: a review. Biomaterials. 2016;83:127-141. doi: 10.1016/j.biomaterials.2016.01.012
- Lacroix D. Biomechanical aspects of bone repair. In: Josep AP, Serena MB, Damien L, Antonio M, eds. Bone Repair Biomaterials. Sawston, Cambridge: Woodhead Publishing; 2009:106-118. doi: 10.1533/9781845696610.1.106
- Jiawei F, Bo L, Zhiwei L, Jianzhong F. Isotropic octet-truss lattice structure design and anisotropy control strategies for implant application. Mater Des. 2021;203:109595. doi: 10.1016/j.matdes.2021.109595
- Jianfeng K, Enchun D, Dichen L, Shuangpeng D, Chen Z, Ling W. Anisotropy characteristics of microstructures for bone substitutes and porous implants with application of additive manufacturing in orthopaedic. Mater Des. 2020;191:108608. doi: 10.1016/j.matdes.2020.108608
- Marie-Michèle G, Sofiane B, Sofiane G, Rémi D, Pierre C, Pierre W. Additive manufacturing of biomaterials for bone tissue engineering – a critical review of the state of the art and new concepts. Prog Mater Sci. 2022;130:100963. doi: 10.1016/j.pmatsci.2022.100963
- Zhang X, Leary M, Tang H, Song T, Qian M. Selective electron beam manufactured Ti-6Al-4V lattice structures for orthopedic implant applications: current status and outstanding challenges. Curr Opin Solid State Mater Sci. 2018;22(3):75-99. doi: 10.1016/j.cossms.2018.05.002
- Bobbert F, Lietaert K, Eftekhari AA, et al. Additively manufactured metallic porous biomaterials based on minimal surfaces: a unique combination of topological, mechanical, and mass transport properties. Acta Biomater. 2017;53:572-584. doi: 10.1016/j.actbio.2017.02.024
- Alabort E, Barba D, Reed RC. Design of metallic bone by additive manufacturing. Scr Mater. 2019;164:110-114. doi: 10.1016/j.scriptamat.2019.01.022
- Chernyshikhin SV, Mahato B, Shiverskii AV, et al. In-plane measurements and computational fluid dynamics prediction of permeability for biocompatible NiTi gyroid scaffolds fabricated via laser powder bed fusion. Int J Bioprint. 2024;10(1):0119. doi: 10.36922/ijb.0119
- Zhu H, Lin Z, Luan Q, et al. Angiogenesis-promoting composite TPMS bone tissue engineering scaffold for mandibular defect regeneration. Int J Bioprint. 2024;10(1):0153. doi: 10.36922/ijb.0153
- Noroozi R, Tatar F, Zolfagharian A, et al. Additively manufactured multi-morphology bone-like porous scaffolds: experiments and micro-computed tomography-based finite element modeling approaches. Int J Bioprint. 2022;8(3). doi: 10.18063/ijb.v8i3.556
- 16. Lijun X, Xiao X, Genzhu F, Shi L, Weidong S, Zhaoxiu J. Compressive performance and energy absorption of additively manufactured metallic hybrid lattice structures. Int J Mech Sci. 2022;219:107093. doi: 10.1016/j.ijmecsci.2022.107093
- White BC, Garland A, Alberdi R, Boyce BL. Interpenetrating lattices with enhanced mechanical functionality. Addit Manuf. 2021;38. doi: 10.1016/j.addma.2020.101741
- Zhao M, Li X, Zhang DZ, Zhai W. TPMS-based interpenetrating lattice structures: design, mechanical properties and multiscale optimization. Int J Mech Sci. 2023;244. doi: 10.1016/j.ijmecsci.2022.108092
- Li X, Yu X, Chua JW, Lee HP, Ding J, Zhai W. Microlattice metamaterials with simultaneous superior acoustic and mechanical energy absorption. Small. 2021;17(24):2100336. doi: 10.1002/smll.202100336
- Fratzl P. Biomimetic materials research: what can we really learn from nature’s structural materials? J R Soc Interface. 2007;4(15):637-642. doi: 10.1098/rsif.2007.0218
- Siddique SH, Hazell PJ, Wang H, Escobedo JP, Ameri AAH. Lessons from nature: 3D printed bio-inspired porous structures for impact energy absorption – a review. Addit Manuf. 2022;58. doi: 10.1016/j.addma.2022.103051
- Li Z, Yang H, Li P, Liu J, Wang J, Xu Y. Fruit biomechanics based on anatomy: a review. Int Agrophys. 2013;27(1): 97-106. doi: 10.2478/v10247-012-0073-z
- Tancogne-Dejean T, Mohr D. Elastically-isotropic truss lattice materials of reduced plastic anisotropy. Int J Solids Struct. 2018;138:24-39. doi: 10.1016/j.ijsolstr.2017.12.025
- Li X, Tan YH, Wang P, Su X, Willy HJ, Herng TS, et al. Metallic microlattice and epoxy interpenetrating phase composites: experimental and simulation studies on superior mechanical properties and their mechanisms. Compos Part A Appl Sci Manuf. 2020;135:105934. doi: 10.1016/j.compositesa.2020.105934
- Yu T, Li X, Zhao M, et al. Truss and plate hybrid lattice structures: simulation and experimental investigations of isotropy, large-strain deformation, and mechanisms. Mater Today Commun. 2023:106344. doi: 10.1016/j.mtcomm.2023.106344
- Ferng Y-M, Lin K-Y. Investigating effects of BCC and FCC arrangements on flow and heat transfer characteristics in pebbles through CFD methodology. Nucl Eng Des. 2013;258:66-75. doi: 10.1016/j.nucengdes.2013.02.009
- Zhao M, Li X, Zhang DZ, Zhai W. Design, mechanical properties and optimization of lattice structures with hollow prismatic struts. Int J Mech Sci. 2023;238. doi: 10.1016/j.ijmecsci.2022.107842
- Zhou J, Xiong S, Liu M, et al. Study on the influence of scaffold morphology and structure on osteogenic performance. Front Bioeng Biotechnol. 2023;11. doi: 10.3389/fbioe.2023.1127162
- Kolken HMA, Callens S, Leeflang M, Mirzaali MJ, Zadpoor A. Merging strut-based and minimal surface meta-biomaterials: decoupling surface area from mechanical properties. Addit Manuf. 2022;52:102684. doi: 10.1016/j.addma.2022.102684
- Li X, Zhao M, Yu X, et al. Multifunctional and customizable lattice structures for simultaneous sound insulation and structural applications. Mater Des. 2023:112354. doi: 10.1016/j.matdes.2023.112354
- Li J, Qin L, Yang K, et al. Materials evolution of bone plates for internal fixation of bone fractures: a review. J Mater Sci Technol. 2020;36:190-208. doi: 10.1016/j.jmst.2019.07.024
- Falkowska A, Seweryn A, Skrodzki M. Strength properties of a porous titanium alloy Ti6Al4V with diamond structure obtained by laser power bed fusion (LPBF). Materials. 2020;13(22):5138. doi: 10.3390/ma13225138
- Lempert GD, Tsour A. Reduction of static friction between surfaces of Ti-6Al-4V and between surfaces of Ti-6Al-4V and Al-7075. Surf Coat Technol. 1992;52(3):291-295. doi: 10.1016/0257-8972(92)90029-A
- Chai G, Manikandan P, Li X. A numerical study on high velocity impact behavior of titanium based fiber metal laminates. J Compos Sci. 2018;2(4):62. doi: 10.3390/jcs2040062
- Goldstein SA. The mechanical properties of trabecular bone: dependence on anatomic location and function. J Biomech. 1987;20(11-12):1055-1061. doi: 10.1016/0021-9290(87)90023-6
- Jae Young R, Richard BA, Charles HT. Young’s modulus of trabecular and cortical bone material: ultrasonic and microtensile measurements. J Biomech. 1993;26(2): 111-119. doi: 10.1016/0021-9290(93)90042-D
- Murphy CM, O’Brien FJ. Understanding the effect of mean pore size on cell activity in collagen-glycosaminoglycan scaffolds. Cell Adh Migr. 2010;4(3):377-381. doi: 10.4161/cam.4.3.11747
- Ashby MF. The properties of foams and lattices. Phil Trans R Soc. 2006;364(1838):15-30. doi: 10.1098/rsta.2005.1678
- Deshpande VS, Ashby MF, Fleck NA. Foam topology: bending versus stretching dominated architectures. Acta Mater. 2001;49(6):1035-1040. doi: 10.1016/S1359-6454(00)00379-7
- Perez RA, Mestres G. Role of pore size and morphology in musculo-skeletal tissue regeneration. Mater Sci Eng C. 2016;61:922-939. doi: 10.1016/j.msec.2015.12.087
- Foster AL, Moriarty TF, Zalavras C, et al. The influence of biomechanical stability on bone healing and fracture-related infection: the legacy of Stephan Perren. Injury. 2021;52(1):43-52. doi: 10.1016/j.injury.2020.06.044