An integrated in silico–in vitro approach for bioprinting core–shell bioarchitectures
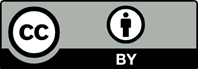
Biological tissues possess a high degree of structural complexity characterized by curvature and stratification of different tissue layers. Despite recent advances in in vitro technology, current engineering solutions do not comprise both of these features. In this paper, we present an integrated in silico–in vitro strategy for the design and fabrication of biological barriers with controlled curvature and architecture. Analytical and computational tools combined with advanced bioprinting methods are employed to optimize living inks for bioprinting-structured core–shell constructs based on alginate. A finite element model is used to compute the hindered diffusion and crosslinking phenomena involved in the formation of core–shell structures and to predict the width of the shell as a function of material parameters. Constructs with a solid alginate-based shell and a solid, liquid, or air core can be reproducibly printed using the workflow. As a proof of concept, epithelial cells and fibroblasts were bioprinted respectively in a liquid core (10 mg/mL Pluronic) and in a solid shell (20 mg/mL alginate plus 20 mg/mL gelatin, used for providing the cells with adhesive moieties). These constructs had a roundness of 97.6% and an average diameter of 1500 ±136 μm. Moreover, their viability was close to monolayer controls (74.12% ± 22.07%) after a week in culture, and the paracellular transport was twice that of cellfree constructs, indicating cell polarization.
Gómez-Gálvez P, Anbari S, Escudero LM, et al., 2021, Mechanics and self-organization in tissue development. Semin Cell Dev Biol, 120: 147–159. https://doi.org/10.1016/j.semcdb.2021.07.003
Moysidou CM, Barberio C, Owens RM, 2021, Advances in engineering human tissue models. Front Bioeng Biotechnol, 8: 620962. https://doi.org/10.3389/fbioe.2020.620962
Nossa R, Costa J, Cacopardo L, et al., 2021, Breathing in vitro: Designs and applications of engineered lung models. J Tissue Eng, 12: 1–28. https://doi.org/10.1177/20417314211008696
Cacopardo L, Costa J, Giusti S, et al., 2019, Real-time cellular impedance monitoring and imaging of biological barriers in a dual-flow membrane bioreactor. Biosens Bioelectron, 140: 111340. https://doi.org/10.1016/j.bios.2019.111340
Costa J, Ahluwalia A, 2019, Advances and current challenges in intestinal in vitro model engineering: A digest. Front Bioeng Biotechnol, 7: 144. https://doi.org/10.3389/fbioe.2019.00144
de Melo BAG, Benincasa JC, Cruz EM, et al., 2021, 3D culture models to study SARS-CoV-2 infectivity and antiviral candidates: From spheroids to bioprinting. Biomed J, 44(1): 31–42. https://doi.org/10.1016/j.bj.2020.11.009
Callens SJP, Uyttendaele RJC, Fratila-Apachitei LE, et al., 2020, Substrate curvature as a cue to guide spatiotemporal cell and tissue organization. Biomaterials, 232: 119739. https://doi.org/10.1016/j.biomaterials.2019.119739
Arumugasaamy N, Navarro J, Kent Leach J, et al., 2019, In vitro models for studying transport across epithelial tissue barriers. Ann Biomed Eng, 47(1): 1–21. https://doi.org/10.1007/s10439-018-02124-w
Charwat V, Egger D, 2018, The third dimension in cell culture: From 2D to 3D culture formats, in Cell Culture Technology. Learning Materials in Biosciences, Springer, Cham, 75–90. https://doi.org/10.1007/978-3-319-74854-2_5
Harmand N, Hénon S, 2020, 3D shape of epithelial cells on curved substrates. Phys Rev X, 11(3): 031028(17). https://doi.org/10.1103/PhysRevX.11.031028
Rosellini A, Freer G, Quaranta P, et al., 2019, Enhanced in vitro virus expression using 3-dimensional cell culture spheroids for infection. J Virol Methods, 265: 99–104. https://doi.org/10.1016/j.jviromet.2018.12.017
Lee JL, Streuli CH, 2014, Integrins and epithelial cell polarity. J Cell Sci, 127(25): 3217–3225. https://doi.org/10.1242/jcs.146142
Samy KE, Levy ES, Phong K, et al., 2019, Human intestinal spheroids cultured using sacrificial micromolding as a model system for studying drug transport. Sci Rep, 9(1): 1–12. https://doi.org/10.1038/s41598-019-46408-0
Abdul L, Rajasekar S, Lin DSY, et al., 2020, Deep-LUMEN assay-human lung epithelial spheroid classification from brightfield images using deep learning. Lab Chip, 20(24): 4623–4631. https://doi.org/10.1039/D0LC01010C
Mithal A, Capilla A, Heinze D, et al., 2020, Generation of mesenchyme free intestinal organoids from human induced pluripotent stem cells. Nat Commun, 11(1): 215. https://doi.org/10.1038/s41467-019-13916-6
Lu T, Cao Y, Zhao P, et al., 2021, Organoid: A powerful tool to study lung regeneration and disease. Cell Regeneration, 10(1): 1–10. https://doi.org/10.1186/s13619-021-00082-8
Laselva O, Conese M, 2021, Three-dimensional airway spheroids and organoids for cystic fibrosis research. J Respir, 1(4): 229–247. https://doi.org/10.3390/jor1040022
Mollaki V, 2021, Ethical challenges in organoid use. BioTech, 10(13): 12. https://doi.org/10.3390/biotech10030012
Garreta E, Kamm RD, Chuva de Sousa Lopes SM, et al., 2021, Rethinking organoid technology through bioengineering. Nat Mater, 20(2): 145–155. https://doi.org/10.1038/s41563-020-00804-4
Fabbri R, Cacopardo L, Ahluwalia A, et al., 2023, Advanced 3D models of human brain tissue using neural cell lines: State-of-the-art and future prospects. Cells, 12(8): 1181. https://doi.org/10.3390/cells12081181
Mobaraki M, Ghaffari M, Yazdanpanah A, et al., 2020, Bioinks and bioprinting: A focused review. Bioprinting, 18: e00080. https://doi.org/10.1016/j.bprint.2020.e00080
Ahmad Raus R, Wan Nawawi WMF, Nasaruddin RR, 2021, Alginate and alginate composites for biomedical applications. Asian J Pharm Sci, 16(3): 280–306. https://doi.org/10.1016/j.ajps.2020.10.001
Choi DH, Park CH, Kim IH, et al., 2010, Fabrication of core-shell microcapsules using PLGA and alginate for dual growth factor delivery system. J Control Release, 147(2): 193–201. https://doi.org/10.1016/j.jconrel.2010.07.103
Duarte ARC, Mano F, Reis RL, et al., 2014, Microfluidic production of per fluorocarbon-alginate core − shell microparticles for ultrasound therapeutic applications. Langmuir, 30(41): 12391–99. https://doi.org/10.1021/la502822v
Yu L, Sun Q, Hui Y, et al., 2019, Microfluidic formation of core-shell alginate microparticles for protein encapsulation and controlled release. J Colloid Interface Sci, 539: 497–503. https://doi.org/10.1016/j.jcis.2018.12.075
Kamperman T, Trikalitis VD, Karperien M, et al., 2018, Ultrahigh-throughput production of monodisperse and multifunctional janus microparticles using in-air microfluidics. ACS Appl Mater Interfaces, 10(28): 23433–23438. https://doi.org/10.1021/acsami.8b05227
Agarwal P, Choi JK, Huang H, et al., 2015, A biomimetic core-shell platform for miniaturized 3D cell and tissue engineering. Part Part Syst Charact, 32(8): 809–816. https://doi.org/10.1002/ppsc.201500025
Wang H, Liu H, Liu H, et al., 2019, One-step generation of core–shell gelatin methacrylate (GelMA) microgels using a droplet microfluidic system. Adv Mater Technol, 4(6): 1–10. https://doi.org/10.1002/admt.201800632.
Wilkes ED, Phillips SD, Basaran OA, 1999, Computational and experimental analysis of dynamics of drop formation. Phys Fluids, 11(12): 3577–3598. https://doi.org/10.1063/1.870224
Hajikhani A, Wriggers P, Marino M, 2021, Chemo-mechanical modelling of swelling and crosslinking reaction kinetics in alginate hydrogels: A novel theory and its numerical implementation. J Mech Phys Solids, 153: 104476. https://doi.org/10.1016/j.jmps.2021.104476
Hajikhani A, Scocozza F, Conti M, et al., 2019, Experimental characterization and computational modeling of hydrogel cross-linking for bioprinting applications. Int J Artif Organs, 42(10): 548–557. https://doi.org/10.1177/0391398819856024
Pluen A, Netti PA, Jain RK, et al., 1999, Diffusion of macromolecules in agarose gels: Comparison of linear and globular configurations. Biophys J, 77(1): 542–552. https://doi.org/10.1016/S0006-3495(99)76911-0
Tirella A, Magliaro C, Penta M, et al., 2014, Sphyga: A multiparameter open source tool for fabricating smart and tunable hydrogel microbeads. Biofabrication, 6(2): 025009. DOI 10.1088/1758-5082/6/2/025009 https://doi.org/10.1088/1758-5082/6/2/025009
Frost TS, Jiang L, Lynch RM, et al., 2019, Permeability of epithelial/endothelial barriers in transwells and microfluidic bilayer devices. Micromachines (Basel), 10(8): 8:553. https://doi.org/10.3390/mi10080533
Costa J, Almonti V, Cacopardo L, et al., 2020, Investigating curcumin/intestinal epithelium interaction in a millifluidic bioreactor. Bioengineering, 7(3): 100. https://doi.org/10.3390/bioengineering7030100
Matricardi P, Pontoriero M, Coviello T, et al., 2008, In situ cross-linkable novel alginate-dextran methacrylate IPN hydrogels for biomedical applications: Mechanical characterization and drug delivery properties. Biomacromolecules, 9(7): 2014–2020. https://doi.org/10.1021/bm800252c
Bharatiya B, Ghosh G, Aswal VK, et al., 2010, Effect of n-Hexanol and n-Hexylamine on the micellar solutions of pluronic F127 and P123 in water and 1M NaCl. J Dispers Sci Technol, 31(5): 660–667, DOI: 10.1080/01932690903212867 http://dx.doi.org/10.1080/01932690903212867
White ES, 2015, Lung extracellular matrix and fibroblast function. Ann Am Thorac Soc, 12(1): 30–33. https://doi.org/10.1513/AnnalsATS.201406-240MG
Ushakumary MG, Riccetti M, Perl AKT, 2021, Resident interstitial lung fibroblasts and their role in alveolar stem cell niche development, homeostasis, injury, and regeneration. Stem Cells Transl Med, 10(7): 1021–1032. https://doi.org/10.1002/sctm.20-0526
Cacopardo L, Ahluwalia A, 2021, Engineering and monitoring 3D cell constructs with time-evolving viscoelasticity for the study of liver fibrosis in vitro. Bioengineering, 8(8): 106. https://doi.org/10.3390/bioengineering8080106
Cacopardo L, Guazzelli N, Ahluwalia A, 2022, Characterizing and engineering biomimetic materials for viscoelastic mechanotransduction studies. Tissue Eng Part B Rev, 28(4): 912–925. https://doi.org/10.1089/ten.teb.2021.0151
Mastrorocco A, Cacopardo L, Lamanna D, et al., 2021, Bioengineering approaches to improve in vitro performance of prepubertal lamb oocytes. Cells, 10(6): 1458. https://doi.org/10.3390/cells10061458
Pinto B, Henriques AC, Silva PMA, et al., 2020, Three-dimensional spheroids as in vitro preclinical models for cancer research. Pharmaceutics, 12(12): 1–38. https://doi.org/10.3390/pharmaceutics12121186
Visco V, Bava FA, D’Alessandro, F, et al., 2009, Human colon fibroblasts induce differentiation and proliferation of intestinal epithelial cells through the direct paracrine action of keratinocyte growth factor. J Cell Physiol, 220(1): 204– 213. https://doi.org/10.1002/jcp.21752
Roulis M, Flavell, RA, 2016, Fibroblasts and myofibroblasts of the intestinal lamina propria in physiology and disease. Differentiation, 92(3): 116–131. https://doi.org/10.1016/j.diff.2016.05.002