Design and biomechanical analysis of patient-specific porous tantalum prostheses for knee joint revision surgery
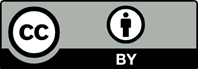
Artificial joint revision surgery, as an increasingly common surgery in orthopedics, often requires patient-specific prostheses to repair the bone defect. Porous tantalum is a good candidate due to its excellent abrasion and corrosion resistance and good osteointegration. Combination of 3D printing technology and numerical simulation is a promising strategy to design and prepare patient-specific porous prostheses. However, clinical design cases have rarely been reported, especially from the viewpoint of biomechanical matching with the patient’s weight and motion and specific bone tissue. This work reports a clinical case on the design and mechanical analysis of 3D-printed porous tantalum prostheses for the knee revision of an 84-year-old male patient. Particularly, standard cylinders of 3D-printed porous tantalum with different pore size and wire diameters were first fabricated and their compressive mechanical properties were measured for following numerical simulation. Subsequently, patientspecific finite element models for the knee prosthesis and the tibia were constructed from the patient’s computed tomography data. The maximum von Mises stress and displacement of the prostheses and tibia and the maximum compressive strain of the tibia were numerically simulated under two loading conditions by using finite element analysis software ABAQUS. Finally, by comparing the simulated data to the biomechanical requirements for the prosthesis and the tibia, a patient-specific porous tantalum knee joint prosthesis with a pore diameter of 600 μm and a wire diameter of 900 μm was determined. The Young’s modulus (5719.32 ± 100.61 MPa) and yield strength (172.71 ± 1.67 MPa) of the prosthesis can produce both sufficient mechanical support and biomechanical stimulation to the tibia. This work provides a useful guidance for designing and evaluating a patient-specific porous tantalum prosthesis.
1. Woiczinski M, Steinbrück A, Weber P, et al., 2015, Development and validation of a weight-bearing finite element model for total knee replacement. Comput Methods Biomech Biomed Eng, 19(10):1033–1045. https://doi.org/10.1080/10255842.2015.1089534
2. Grelsamer RP, 2007, Applications of porous tantalum in total hip arthroplasty. J Am Acad Orthop Surg, 14(12):646–655. https://doi.org/10.1016/j.jse.2005.09.001
3. Matsuno H, Yokoyama A, Watari F, et al., 2001, Biocompatibility and osteogenesis of refractory metal implants, titanium, hafnium, niobium, tantalum and rhenium. Biomaterials, 22(11):1253–1262. https://doi.org/10.1016/S0142-9612(00)00275-1
4. Zou X, Li H, Bünger M, et al., 2004, Bone ingrowth characteristics of porous tantalum and carbon fiber interbody devices: An experimental study in pigs. Spine J, 4(1):99–105. https://doi.org/10.1016/S1529-9430(03)00407-8
5. Bobyn JD, Stackpool GJ, Hacking SA, et al., 1999, Characteristics of bone ingrowth and interface mechanics of a new porous tantalum biomaterial. J Bone Joint Surg Br, 81(5):907–914. https://doi.org/10.1302/0301-620X.81B5.9283
6. Rossi SMP, Perticarini L, Ghiara M, et al., 2022, High survival rate at mid-term follow up of porous tantalum cones for bone defects in revision total knee replacement: A 3–11 years follow up report. Knee, 35(2022):175–182. https://doi.org/10.1016/j.knee.2022.03.007
7. Huang G, Pan S, Qiu J, 2021, The clinical application of porous tantalum and its new development for bone tissue engineering. Materials, 14(10):2647. https://doi.org/10.3390/ma14102647
8. Kamath AF, Gee AO, Nelson CL, et al., 2012, Porous tantalum patellar components in revision total knee arthroplasty minimum 5-year follow-up. J Arthroplast, 27(1):82–87. https://doi.org/10.1016/j.arth.2011.04.024
9. Kamath AF, Lee GC, Sheth NP, et al., 2011, Prospective results of uncemented tantalum monoblock tibia in total knee arthroplasty: Minimum 5-year follow-up in patients younger than 55 years. J Arthroplast, 26(8):1390–1395. https://doi.org/10.1016/j.arth.2011.06.030
10. Howard JL, Kudera J, Lewallen DG, et al., 2011, Early results of the use of tantalum femoral cones for revision total knee arthroplasty. J Bone Joint Surg Am, 93(5):478–484. https://doi.org/10.2106/JBJS.I.01322
11. Unger AS, Duggan JP, 2011, Midterm results of a porous tantalum monoblock tibia component clinical and radiographic results of 108 knees. J Arthroplast, 26(6):855–860. https://doi.org/10.1016/j.arth.2010.08.017
12. Kaplan RB, 1994, Open cell tantalum structures for cancellous bone implants and cell and tissue receptors. EP, EP0560279 B1.
13. Thijs L, Sistiaga MM, Wauthle R, et al., 2013, Strong morphological and crystallographic texture and resulting yield strength anisotropy in selective laser melted tantalum. Acta Biomater, 61(12):4657–4668. https://doi.org/10.1016/j.actamat.2013.04.036
14. Song C, Deng Z, Zou Z, et al., 2022, Pure tantalum manufactured by laser powder bed fusion: Influence of scanning speed on the evolution of microstructure and mechanical properties. Int J Refract Metals Hard Mater, 107(2022):105882. https://doi.org/10.1016/j.ijrmhm.2022.105882
15. Gao H, Jin X, Yang J, et al., 2021, Porous structure and compressive failure mechanism of additively manufactured cubic-lattice tantalum scaffolds. Mater Today Adv, 12(2021):100183. https://doi.org/10.1016/j.mtadv.2021.100183
16. Tang HP, Yang K, Jia L, et al., 2020, Tantalum bone implants printed by selective electron beam manufacturing (SEBM) and their clinical applications. J Miner Metals Mater Soc, 72(3):1016–1021. https://doi.org/10.1007/s11837-020-04016-8
17. Risse L, Woodcock S, Brüggemann J, et al., 2022, Stiffness optimization and reliable design of a hip implant by using the potential of additive manufacturing processes. BioMed Eng OnLine, 21(1):23. https://doi.org/10.1186/s12938-022-00990-z
18. Kharmanda G, Gowid S, Mahdi E, et al., 2020, Efficient system reliability-based design optimization study for replaced hip prosthesis using new optimized anisotropic bone formulations. Materials, 13(2):362. https://doi.org/10.3390/ma13020362
19. Frost HM, 2004, A 2003 update of bone physiology and Wolff ’s law for clinicians. Angle Orthod, 74(1):3–15. https://doi.org/10.1043/0003-3219(2004)0742.0.CO;2
20. Cowin SC, 2002, Mechanosensation and fluid transport in living bone. J Musculoskelet Neuronal Interact, 2(3):256–260.
21. Zupancic Cepic L, Frank M, Reisinger A, et al., 2022, Biomechanical finite element analysis of short-implant-supported, 3-unit, fixed CAD/CAM prostheses in the posterior mandible. Int J Implant Dent, 8(1):8. https://doi.org/10.1186/s40729-022-00404-8
22. Liu B, Li X, Qiu W, et al., 2022, Mechanical distribution and new bone regeneration after implanting 3D printed prostheses for repairing metaphyseal bone defects: A finite element analysis and prospective clinical study. Front Bioeng Biotechnol, 10:921545. https://doi.org/10.3389/fbioe.2022.921545
23. Lemos CAA, Verri FR, Santiago Junior JF, et al., 2018, Splinted and nonsplinted crowns with different implant lengths in the posterior maxilla by three-dimensional finite element analysis. J Healthc Eng, 2018:1–7. https://doi.org/10.1155/2018/3163096
24. Silva LS, Verri FR, Lemos CAA, et al., 2021, Biomechanical effect of an occlusal device for patients with an implant-supported fixed dental prosthesis under parafunctional loading: A 3D finite element analysis. J Prosthet Dent, 126(2):223.e1–223.e8. https://doi.org/10.1016/j.prosdent.2021.04.024
25. Mikushev VM, Samarkin AI, Khomutova AS, 2021, Finite element simulations of stresses in bone implants made by three-dimensional printing. IOP Conf Series: Mater Sci Eng, 1117(1):12006. https://doi.org/10.1088/1757-899X/1117/1/012006
26. Mirulla AI, Di Paolo S, Di Simone F, et al., 2020, Biomechanical analysis of two types of osseointegrated transfemoral prosthesis. Appl Sci Basel, 10(22):8263. https://doi.org/10.3390/app10228263
27. Uğur L, Ozturk B, Erzincanli F, 2022, Reduction of stress variations on sections (ROSVOS) for a femoral component. Iran J Sci Technol Transact Mech Eng, 46(1):237–252. https://doi.org/10.1007/s40997-020-00418-w
28. Öztürk B, Erzincanli F, 2019, Development of femoral component design geometry by using DMROVAS (design method requiring optimum volume and safety). Eng Comput, 37(2): 682–704. https://doi.org/10.1108/EC-03-2019-0077
29. Francisco AV, Raquel CO, Luis-Guillermo OL, et al., 2019, Influence of bone quality on the mechanical interaction between implant and bone: A finite element analysis. J Dent, 88:103161. https://doi.org/10.1016/j.jdent.2019.06.008
30. Cheong VS, Mumith A, Coathup M, et al., 2020, Bone remodeling in additive manufactured porous implants changes the stress distribution, in Health Monitoring of Structural and Biological Systems XIV; International Society for Optics and Photonics, Bellingham, WA, USA. https://doi.org/10.1117/12.2558093
31. Zysset PK, Guo XE, Hoffler CE, et al., 1999, Elastic modulus and hardness of cortical and trabecular bone lamellae measured by nanoindentation in the human femur. J Biomech, 32(10):1005–1012. https://doi.org/10.1016/S0021-9290(99)00111-6
32. Rho JY, Ashman RB, Turner CH, 1993, Young’s modulus of trabecular and cortical bone material: ultrasonic and microtensile measurements. J Biomech, 26(2):111–119. https://doi.org/10.1016/0021-9290(93)90042-D
33. Arabnejad S, Burnett JR, Pura JA, et al., 2016, High-strength porous biomaterials for bone replacement: a strategy to assess the interplay between cell morphology, mechanical properties, bone ingrowth and manufacturing constraints. Acta Biomater, 30(2016):345–356. https://doi.org/10.1016/j.actbio.2015.10.048
34. Mckown S, Shen Y, Brookes WK, et al., 2008, The quasi-static and blast loading response of lattice structures. Int J Impact Eng, 35(8):795–810. https://doi.org/10.1016/j.ijimpeng.2007.10.005
35. Yan C, Liang H, Raymont D, et al., 2012, Evaluations of cellular lattice structures manufactured using selective laser melting. Int J Mach Tools Manuf, 62(2012):32–38. https://doi.org/10.1016/j.ijmachtools.2012.06.002
36. Bayraktar HH, Morgan EF, Niebur GL, et al., 2004, Comparison of the elastic and yield properties of human femoral trabecular and cortical bone tissue. J Biomech, 37(1):27–35. https://doi.org/10.1016/S0021-9290(03)00257-4
37. Levine BR, Sporer S, Poggie RA, et al., 2006, Experimental and clinical performance of porous tantalum in orthopedic surgery. Biomaterials, 27(27):4671–4681. https://doi.org/10.1016/j.biomaterials.2006.04.041
38. Kowalczyk P, 2006, Orthotropic properties of cancellous bone modelled as parameterized cellular material. Comput Methods Biomech Biomed Eng, 9(3):135–147. https://doi.org/10.1080/10255840600751473
39. Liu L, Shi Q, Chen Q, et al., 2019, Mathematical modeling of bone in-growth into undegradable porous periodic scaffolds under mechanical stimulus. J Tissue Eng, 10:1–13. https://doi.org/10.1177/2041731419827167
40. Palomares KTS, Gleason RE, Mason ZD, et al., 2009, Mechanical stimulation alters tissue differentiation and molecular expression during bone healing. J Orthop Res, 27(9):1123–1132. https://doi.org/10.1002/jor.20863
41. Liu L, Duan J, Shi Q, et al., 2020, Mechanical effect on the evolution of bone formation during bone ingrowth into a 3D-printed Ti-alloy scaffold. Mater Lett, 273(2020): 127921. https://doi.org/10.1016/j.matlet.2020.127921
42. Carter DR, 1987, Mechanical loading history and skeletal biology. J Biomech, 20(11–12):1095–1109. https://doi.org/10.1016/0021-9290(87)90027-3
43. Betts DC, Müller R, 2014, Mechanical regulation of bone regeneration: Theories, models, and experiments. Front Endocrinol, 5:211. https://doi.org/10.3389/fendo.2014.00211