Monolayer heterojunction interactive hydrogels for high-freedom 4D shape reconfiguration by two-photon polymerization
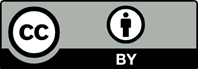
Mimicking natural botanical/zoological systems has revolutionarily inspired fourdimensional (4D) hydrogel robotics, interactive actuators/machines, automatic biomedical devices, and self-adaptive photonics. The controllable high-freedom shape reconfiguration holds the key to satisfying the ever-increasing demands. However, miniaturized biocompatible 4D hydrogels remain rigorously stifled due to current approach/material limits. In this research, we spatiotemporally program micro/ nano (μ/n) hydrogels through a heterojunction geometric strategy in femtosecond laser direct writing (fsLDW). Polyethylene incorporated N-isopropylacrylamide as programmable interactive materials here. Dynamic chiral torsion, site-specific mutation, anisotropic deformation, selective structural coloration of hydrogel nanowire, and spontaneous self-repairing as reusable μ/n robotics were identified. Hydrogel-materialized monolayer nanowires operate as the most fundamental block at nanometric accuracy to promise high freedom reconfiguration and high force-to-weight ratio/bending curvature under tight topological control. Taking use of this biomimetic fsLDW, we spatiotemporally constructed several in/out-plane selfdriven hydrogel grippers, diverse 2D-to-3D transforming from the same monolayer shape, responsive photonic crystal, and self-clenched fists at μ/n scale. Predictably, the geometry-modulable hydrogels would open new access to massively-reproducible robotics, actuators/sensors for microenvironments, or lab-on-chip devices.
1. Sun HL, Klok HA, Zhong ZY 2018, Polymers from nature and for nature. Biomacromolecules, 19: 1697–1700. https://doi.org/10.1021/acs.biomac.8b00830
2. Xu F, Fu CB, Yang YF, 2020, Water affects morphogenesis of growing aquatic plant leaves. Phys Rev Lett, 124: 038003. https://doi.org/10.1103/physrevlett.124.038003
3. Liang H, Mahadevan L, 2011, Growth, geometry, and mechanics of a blooming lily. Proc Natl Acad Sci, 108: 5516–5521. https://doi.org/10.1073/pnas.1007808108
4. Hu Y, Wang Z, Jin D, et al., 2019, Botanical-inspired 4D printing of hydrogel at the microscale. Adv Funct Mater, 30: 1907377. https://doi.org/10.1002/adfm.201907377
5. Miao SD, Castro N, Nowicki M, et al., 2017, 4D printing of polymeric materials for tissue and organ regeneration. Mater Today, 20: 577–591. https://doi.org/10.1016/j.mattod.2017.06.005
6. Bastola A, Rodriguez NJ, Behl M, et al., 2021, Cactus-inspired design principles for soft robotics-based on 3D printed hydrogel-elastomer systems. Mater Design, 202: 109515. https://doi.org/10.1016/j.matdes.2021.109515
7. Almeida AC, Canejo J, Fernandes S, et al., 2018, Cellulose-based biomimetics and their applications. Adv Mater, 30: 1703655. https://doi.org/10.1002/adma.201703655
8. Jeong HY, Lee E, An S, et al., 2020, 3D and 4D printing for optics and metaphotonics. Nanophotonics, 9: 1139–1160. https://doi.org/10.1515/nanoph-2019-0483
9. Wang S, Lee JM, Yeong WY, 2015, Smart hydrogels for 3D bioprinting, Int J Bioprint, 1: 01005.
10. Apsite I, Biswas A, Li Y, et al., 2020, Microfabrication using shape-transforming materials. Adv Funct Mater, 30: 1908028. https://doi.org/10.1002/adfm.201908028
11. Gladman AS, Matsumoto EA, Nuzzo RG, et al., 2016, Biomimetic 4D printing, Nat Mater, 15: 413–418. https://doi.org/10.1038/nmat4544
12. Son H, Byun E, Yoon YJ, et al., 2020, Untethered actuation of hybrid hydrogel gripper via ultrasound. ACS Macro Lett, 9: 1766–1772. https://doi.org/10.1021/acsmacrolett.0c00702
13. Visentin F, Babu SP, Meder F, et al., 2021, Selective stiffening in soft actuators by triggered phase transition of hydrogel‐filled elastomers. Adv Funct Mater, 31: 2101121. https://doi.org/10.1002/adfm.202101121
14. Mishra AK, Pan WY, Giannelis E, et al., 2021, Making bioinspired 3D-printed autonomic perspiring hydrogel actuators. Nat Protoc, 16: 2068–2087. https://doi.org/10.1038/s41596-020-00484-z
15. Adam G, Benouhiba A, Rabenorosoa K, et al., 2021, 4D printing: Enabling technology for microrobotics applications. Adv Intell Syst, 3: 2000216. https://doi.org/10.1002/aisy.202000216
16. McLellan K, Sun YC, Naguib H, 2022, A review of 4D printing: Materials, structures, and designs towards the printing of biomedical wearable devices. Bioprinting, 27: e00217. https://doi.org/10.1016/j.bprint.2022.e00217
17. Xing J, Zheng M, Duan X, 2015, Two-photon polymerization microfabrication of hydrogels: An advanced 3D printing technology for tissue engineering and drug delivery. Chem Soc Rev, 44: 5031–5039. https://doi.org/10.1039/c5cs00278h
18. Jin DD, Chen QY, Huang T, et al., 2020, Four-dimensional direct laser writing of reconfigurable compound micromachines. Mater Today, 32: 19–25. https://doi.org/10.1016/j.mattod.2019.06.002
19. Gardi G, Ceron S, Wang W, et al., 2022, Microrobot collectives with reconfigurable morphologies, behaviors, and functions. Nat Commun, 13: 2239. https://doi.org/10.1038/s41467-022-29882-5
20. Zhuo SY, Zhao ZG, Xie ZX, et al., 2020, Complex multiphase organohydrogels with programmable mechanics toward adaptive soft-matter machines. Sci Adv, 6: eaax1464. https://doi.org/10.1126/sciadv.aax1464
21. Zhao Z, Kuang X, Yuan C, et al., 2018, Hydrophilic/ hydrophobic composite shape-shifting structures. ACS Appl Mater Interf, 10: 19932–19939. https://doi.org/10.1021/acsami.8b02444
22. Wu Y, Hao X, Xiao R, et al., 2019, Controllable bending of bi-hydrogel strips with differential swelling. Acta Mech Solida Sin, 32: 652–662. https://doi.org/10.1007/s10338-019-00106-6
23. Van Hoorick J, 2017, Cross-linkable gelatins with superior mechanical properties through carboxylic acid modification: Increasing the two-photon polymerization potential. Biomacromolecules, 18: 3260–3272. https://doi.org/10.1021/acs.biomac.7b00905
24. Urrios A, Parra-Cabrera C, Bhattacharjee N, et al., 2016, 3D-printing of transparent bio-microfluidic devices in PEG-DA. Lab Chip, 16: 2287–2294. https://doi.org/10.1039/c6lc00153j
25. Dey R, Mukherjee R, Haldar J, 2022, Photo-crosslinked antimicrobial hydrogel exhibiting wound healing ability and curing infections in vivo. Adv Healthc Mater, 5: 2224–2231. https://doi.org/10.1002/adhm.202200536
26. Ceylan H, Yasa IC, Yasa O, et al., 3D-printed biodegradable microswimmer for theranostic cargo delivery and release. ACS Nano, 13: 3353–3362. https://doi.org/10.1021/acsnano.8b09233
27. Tao YF, Lu CC, Deng CS, et al., 2022, Four-dimensional stimuli-responsive hydrogels micro-structured via femtosecond laser additive manufacturing. Micromachines, 13: 32. https://doi.org/10.3390/mi13010032
28. Bauhofer AA, Krödel S, Rys J, et al., 2017, Harnessing photochemical shrinkage in direct laser writing for shape morphing of polymer sheets. Adv Mater, 29: 1703024. https://doi.org/10.1002/adma.201703024
29. Zhang F, Lian MY, Alhadhrami A, et al., 2022, Laccase immobilized on functionalized cellulose nanofiber/alginate composite hydrogel for efficient bisphenol A degradation from polluted water. Adv Compos Hybrid Mater, 5: 1852–1864. https://doi.org/10.1007/s42114-022-00476-5
30. Kong D, EI-Bahy ZM, Algadi H, et al., 2022, Highly sensitive strain sensors with wide operation range from strong MXene-composited polyvinyl alcohol/sodium carboxymethylcellulose double network hydrogel. Adv Compos Hybrid Mater, 5: 1976–1987. https://doi.org/10.1007/s42114-022-00531-1
31. Wu YF, Chen EF, Weng XD, et al., 2022, Conductive polyvinyl alcohol/silver nanoparticles hydrogel sensor with large draw ratio, high sensitivity and high stability for human behavior monitoring. Eng Sci, 18: 113–120.
32. Huang K, Wu YF, Liu JC, et al., 2022, A double-layer carbon nanotubes/polyvinyl alcohol hydrogel with high stretchability and compressibility for human motion detection. Eng Sci, 17: 319–327.
33. Tao YF, Wei CY, Liu JW, et al., 2019, Nanostructured electrically conductive hydrogels via ultrafast laser processing and self-assembly. Nanoscale, 11: 9176–9184. https://doi.org/10.1039/c9nr01230c
34. Yamamoto Y, Kanao K, Arie T, et al., 2015, Air ambient-operated pNIPAM-based flexible actuators stimulated by human body temperature and sunlight. ACS Appl Mater Interf, 7: 11002–11006. https://doi.org/10.1021/acsami.5b02544
35. Forg S, Karbacher A, Ye ZS, et al., 2022, Copolymerization kinetics of dopamine methacrylamide during PNIPAM microgel synthesis for increased adhesive properties. Langmuir, 38: 5275–5285. https://doi.org/10.1021/acs.langmuir.1c02749
36. Wolski L, Ziolek M, 2018, Insight into pathways of methylene blue degradation with H2O2 over mono and bimetallic Nb, Zn oxides. Appl Catal B Environ, 224: 634–647. https://doi.org/10.1016/j.apcatb.2017.11.008
37. Fernández-Pérez A, Marbán G, 2022, Visible light spectroscopic analysis of methylene blue in water. J Appl Spectrosc, 88: 1284–1290. https://doi.org/10.1007/s10812-022-01310-y
38. Özcan M, Cakmakci M, Temize İ, 2020, Smart composites with tunable stress-strain curves. Comput Mech, 65: 375–394. https://doi.org/10.1007/s00466-019-01773-5
39. Yang LX, Yang LB, Lowe RL, 2021, A viscoelasticity model for polymers: Time, temperature, and hydrostatic pressure dependent Young’s modulus and Poisson’s ratio across transition temperatures and pressures. Mech Mater, 157: 103839. https://doi.org/10.1016/j.mechmat.2021.103839
40. Tao YF, Deng CS, Long J, et al., 2022, Multiprocess laser lifting-off for nanostructured semiconductive hydrogels. Adv Mater Interf, 9: 2101250. https://doi.org/10.1002/admi.202101250
41. Tao YF, Ren YP, Wang XJ, et al., 2021, A femtosecond laser-assembled SnO2 microbridge on interdigitated Au electrodes for gas sensing. Mater Lett, 308: 131120. https://doi.org/10.1016/j.matlet.2021.131120
42. Hua J, Björling M, Larsson R, et al., 2022, Friction control of chitosan-Ag hydrogel by silver ion. ES Mater Manuf, 16: 30–36.
43. Kordjazi S, Kamyab K, Hemmatinejad N, 2020, Super-hydrophilic/oleophobic chitosan/acrylamide hydrogel: An efficient water/oil separation filter. Adv Compos Hybrid Mater, 3: 167–176. https://doi.org/10.1007/s42114-020-00150-8
44. Zhao WW, Chen LJ, Hu SM, et al., 2020, Printed hydrogel nanocomposites: Fine-tuning nanostructure for anisotropic mechanical and conductive properties. Adv Compos Hybrid Mater, 3: 315–324. https://doi.org/10.1007/s42114-020-00161-5
45. Wang TR, Wusigale, Kuttappan D, et al., 2021, Polydopamine-coated chitosan hydrogel beads for synthesis and immobilization of silver nanoparticles to simultaneously enhance antimicrobial activity and adsorption kinetics. Adv Compos Hybrid Mater, 4: 696–706. https://doi.org/10.1007/s42114-021-00305-1
46. Liu Z, Wang Y, Ren YY, et al., 2020, Poly(ionic liquid) hydrogel-based anti-freezing ionic skin for a soft robotic gripper. Mater Horiz, 7: 919–927. https://doi.org/10.1039/c9mh01688k
47. Zhang Y, Le X, Jian Y, et al., 2019, 3D fluorescent hydrogel origami for multistage data security protection. Adv Funct Mater, 29: 1905514. https://doi.org/10.1002/adfm.201905514
48. Zhu QY, Du C, Dai YH, et al., 2020, Light-steered locomotion of muscle-like hydrogel by self-coordinated shape change and friction modulation. Nat Commun, 11: 5166. https://doi.org/10.1038/s41467-020-18801-1
49. Capella V, Rivero R, Liaudat A, et al., 2019, Cytotoxicity and bioadhesive properties of poly-N-isopropylacrylamide hydrogel. Heliyon, 5: e01474. https://doi.org/10.1016/j.heliyon.2019.e01474
50. Urzedo AL, Gonçalves MC, Nascimento MH, et al., 2020, Cytotoxicity and antibacterial activity of alginate hydrogel containing nitric oxide donor and silver nanoparticles for topical applications. ACS Biomater Sci Eng, 6: 2117–2134. https://doi.org/10.1021/acsbiomaterials.9b01685
51. Mölzer C, Shankar SP, Masalski V, et al., 2019, TGF-β1- activated Type 2 dendritic cells promote wound healing and induce fibroblasts to express Tenascin C following corneal full-thickness hydrogel transplantation. J Tissue Eng Regen Med, 13: 1507–1517. https://doi.org/10.1002/term.2853
52. Ma XY, Zhong W, Zhao J, et al., 2020,“Self-heating” enabled one-pot synthesis of fluorescent carbon dots. Eng Sci, 9: 44–49.
53. Zhao L, Tallman T, Lin G, 2021, Spatial damage characterization in self-sensing materials via neural network-aided electrical impedance tomography: A computational study. ES Mater Manuf, 12: 78–88. https://doi.org/10.30919/esmm5f919
54. Song TT, Jiang BJ, Li YD, et al., 2021, Self-healing materials: A review of recent developments. ES Mater Manuf, 14: 1–19.
55. Sun DW, Yan JH, Ma XU, et al., 2021, Tribological investigation of self-healing composites containing metal/ polymer microcapsules. ES Mater Manuf,14: 59–72.
56. Zhang DJ, Wang XY, He YX, et al., 2022, Self-restoration of underwater low adhesive superoleophobicity on shape memory polymer arrays. Eng Sci, 17: 343–50.
57. Chen F, Xiao H, Peng ZQ, et al., 2021, Thermally conductive glass fiber reinforced epoxy composites with intrinsic self-healing capability. Adv Compos Hybrid Mater, 4: 1048–1058. https://doi.org/10.1007/s42114-021-00303-3
58. Liu C, Yin Q, Li X, et al., 2021, A waterborne polyurethane-based leather finishing agent with excellent room temperature self-healing properties and wear-resistance. Adv Compos Hybrid Mater, 4: 138–149. https://doi.org/10.1007/s42114-021-00206-3