A Review on Bioinks and their Application in Plant Bioprinting
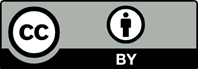
In recent years, the characterization and fabrication methods concerning new bioinks have received much attention, largely because the absence of bioprintable materials has been identified as one of the most rudimentary challenges for rapid advancement in the field of three-dimensional (3D) printing. Bioinks for printing mammalian organs have been rapidly produced, but bioinks in the field of plant science remain sparse. Thus, 3D fabrication of plant parts is still in its infancy due to the lack of appropriate bioink materials, and aside from that, the difficulty in recreating sophisticated microarchitectures that accurately and safely mimic natural biological activities is a concern. Therefore, this review article is designed to emphasize the significance of bioinks and their applications in plant bioprinting.
1. Mobaraki M, Ghaffari M, Yazdanpanah A, et al., 2020, Bioinks and Bioprinting: A Focused Review. Bioprinting, 18:e00080. https://doi.org/10.1016/j.bprint.2020.e00080
2. Donderwinkel I, Van Hest JC, Cameron NR, 2017, Bio-inks for 3D Bioprinting: Recent Advances and Future Prospects. Polym Chem, 8:4451–71.
3. Gopinathan J, Noh I, 2018, Recent Trends in Bioinks for 3D Printing. Biomater Res, 22:11. https://doi.org/10.1186/s40824-018-0122-1
4. Guvendiren M, Molde J, Soares RM, et al., 2016, Designing Biomaterials for 3D Printing. ACS Biomater Sci Eng, 2:1679–93. https://doi.org/10.1021/acsbiomaterials.6b00121
5. Gungor-Ozkerim PS, Inci I, Zhang YS, et al., 2018, Bioinks for 3D Bioprinting: An Overview. Biomater Sci, 6:915–46. https://doi.org/10.1039/c7bm00765e
6. Parak A, Pradeep P, du Toit LC, et al., 2019, Functionalizing Bioinks for 3D Bioprinting Applications. Drug Discov Today, 24:198–205. https://doi.org/10.1016/j.drudis.2018.09.012
7. Mironov V, 2003, Printing Technology to Produce Living Tissue. Expert Opin Biol Ther, 3:701–4. https://doi.org/10.1517/14712598.3.5.701
8. Mironov V, Markwald RR, Forgacs G, 2003, Organ Printing: Self-Assembling Cell Aggregates as “Bioink”. Sci Med, 9: 69–71.
9. Groll J, Burdick JA, Cho DW, et al., 2018, A Definition of Bioinks and their Distinction from Biomaterial Inks. Biofabrication, 11:013001. https://doi.org/10.1088/1758-5090/aaec52
10. Muthukrishnan L, 2021, Imminent Antimicrobial Bioink Deploying Cellulose, Alginate, EPS and Synthetic Polymers for 3D Bioprinting of Tissue Constructs. Carbohydr Polym, 260:117774.
11. Wu D, Yue Y, Tan J, et al., 2018, 3D Bioprinting of Gellan Gum and Poly (Ethylene Glycol) Diacrylate Based Hydrogels to Produce Human-scale Constructs with High-fidelity. Mater Des, 160:486–95. https://doi.org/10.1016/j.matdes.2018.09.040
12. Hu D, Wu D, Huang L, et al., 2018, 3D Bioprinting of Cell-Laden Scaffolds for Intervertebral Disc Regeneration. Mater Lett, 223:219–22.
13. Crapo PM, Gilbert TW, Badylak SF, 2011, An Overview of Tissue and Whole Organ Decellularization Processes. Biomaterials, 32:3233–43. https://doi.org/10.1016/j.biomaterials.2011.01.057
14. Nikolova MP, Chavali MS, 2019, Recent Advances in Biomaterials for 3D Scaffolds: A Review. Bioact Mater, 4:271–92. https://doi.org/10.1016/j.bioactmat.2019.10.005
15. Benwood C, Chrenek J, Kirsch RL, et al., 2021, Natural Biomaterials and their Use as Bioinks for Printing Tissues. Bioengineering, 8:27.
16. Bishop ES, Mostafa S, Pakvasa M, et al., 2017, 3-D Bioprinting Technologies in Tissue Engineering and Regenerative Medicine: Current and Future Trends. Genes Dis, 4:185–95. https://doi.org/10.1016/j.gendis.2017.10.002
17. Jovic TH, Kungwengwe G, Mills AC, et al., 2019, Plant derived Biomaterials: A Review of 3D Bioprinting and Biomedical Applications. Front Mech Eng, 5:19.
18. Wicaksono A, da Silva JA, 2015, Plant Bioprinting: Novel Perspective for Plant Biotechnology. J Plant Dev, 22:135–41.
19. Mehrotra S, Kumar S, Srivastava V, et al., 2019, 3D Bioprinting in Plant Science: An Interdisciplinary Approach. Trends Plant Sci, 25:9–13. https://doi.org/10.1016/j.tplants.2019.10.014
20. Alhazmi HA, Najmi A, Javed SA, et al., 2021, Medicinal Plants and Isolated Molecules Demonstrating Immunomodulation Activity as Potential Alternative Therapies for Viral Diseases Including COVID-19. Front Immunol, 12:637553. https://doi.org/10.3389/fimmu.2021.637553
21. Alamgir M, Uddin SJ, 2010, Recent Advances on the Ethnomedicinal Plants as Immunomodulatory Agents. Ethnomedicine, 37:2.
22. Abood WN, Fahmi I, Abdulla MA, et al., 2014, Immunomodulatory Effect of an Isolated Fraction from Tinospora crispa on Intracellular Expression of INF-γ, IL-6 and IL-8. BMC Complement Altern Med, 14:205. https://doi.org/10.1186/1472-6882-14-205
23. Mishra KP, Ganju L, Sairam M, et al., 2008, A Review of High throughput Technology for the Screening of Natural Products. Biomed Pharmacother, 62:94–8.
24. Schütz K, Placht A, Paul B, et al., 2017, Three-dimensional Plotting of a Cell-laden Alginate/Methylcellulose Blend: Towards Biofabrication of Tissue Engineering Constructs with Clinically Relevant Dimensions. J Tissue Eng Regen Med, 11:1574–87. https://doi.org/10.1002/term.2058
25. Lode A, Krujatz F, Brüggemeier S, et al., 2015, Green Bioprinting: Fabrication of Photosynthetic Algae-laden Hydrogel Scaffolds for Biotechnological and Medical Applications. Eng Life Sci, 15:177–83. https://doi.org/10.1002/elsc.201400205
26. Krujatz F, Lode A, Brüggemeier S, et al., 2015, Green Bioprinting: Viability and Growth Analysis of Microalgae Immobilized in 3D-plotted Hydrogels Versus Suspension Cultures. Eng Life Sci, 15:678–88.
27. Seidel J, Ahlfeld T, Adolph M, et al., 2017, Green Bioprinting: Extrusion-based Fabrication of Plant Cell-laden Biopolymer Hydrogel Scaffolds. Biofabrication, 9:045011. https://doi.org/10.1088/1758-5090/aa8854
28. Brodelius P, 1985, The Potential Role of Immobilization in Plant Cell Biotechnology. Trends Biotechnol, 3:280–5.
29. Singh B, Kaur A, 2014, In Vitro Production of Beneficial Bioactive Compounds From Plants by Cell Immobilization. Biotechnol. Plant Biotechnol, 39:207–36.
30. Beckwith AL, Borenstein JT, Velásquez-García LF, 2021, Tunable Plant-based Materials Via In Vitro Cell Culture using a Zinnia Elegans Model. J Clean Prod, 288:125571.
31. Griffiths M, 2020, A 3D Print Repository for Plant Phenomics. Plant Phenomics, 2020:8640215. https://doi.org/10.34133/2020/8640215
32. Gershlak JR, Hernandez S, Fontana G, et al., 2017, Crossing Kingdoms: Using Decellularized Plants as Perfusable Tissue Engineering Scaffolds. Biomaterials, 125:13–22. https://doi.org/10.1016/j.biomaterials.2017.02.011
33. Ott HC, Matthiesen TS, Goh S, et al., 2008, Perfusion decellularized Matrix: Using Nature’s Platform to Engineer a Bioartificial Heart. Nat Med, 14:213–21. https://doi.org/10.1038/nm1684
34. Lee HJ, Kim YB, Ahn SH, et al., 2015, A New Approach for Fabricating Collagen/ECM-Based Bioinks Using Preosteoblasts and Human Adipose Stem Cells. Adv Healthc Mater, 4:1359–68. https://doi.org/10.1002/adhm.201500193
35. Guyette JP, Charest JM, Mills RW, et al., 2016, Bioengineering Human Myocardium on Native Extracellular Matrix. Circ Res, 118:56–72. https://doi.org/10.1161/CIRCRESAHA.115.306874
36. Kryou C, Leva V, Chatzipetrou M, et al., 2019, Bioprinting for Liver Transplantation. Bioengineering (Basel), 6:95. https://doi.org/10.3390/bioengineering6040095
37. Hospodiuk M, Dey M, Sosnoski D, et al., 2017, The Bioink: A Comprehensive Review on Bioprintable Materials. Biotechnol Adv, 35:217–39. https://doi.org/10.1016/j.biotechadv.2016.12.006
38. Khoshnood N, Zamanian A, 2020, A Comprehensive Review on Scaffold-free Bioinks for Bioprinting. Bioprinting, 19:e00088.
39. Zafar MS, Amin F, Fareed MA, et al., 2020, Biomimetic Aspects of Restorative Dentistry Biomaterials. Biomimetics (Basel), 5:34. https://doi.org/10.3390/biomimetics5030034
40. Norotte C, Marga FS, Niklason LE, et al., 2009, Scaffold-free Vascular Tissue Engineering Using Bioprinting. Biomaterials, 30:5910–7. https://doi.org/10.1016/j.biomaterials.2009.06.034
41. Ovsianikov A, Khademhosseini A, Mironov V, 2018, The Synergy of Scaffold-based and Scaffold-free Tissue Engineering Strategies. Trends Biotechnol, 36:348–57. https://doi.org/10.1016/j.tibtech.2018.01.005
42. Ozbolat IT, 2015, Scaffold-based or Scaffold-free Bioprinting: Competing or Complementing Approaches? J Nanotechnol Eng Med, 6:024701.
43. Shimizu T, Yamato M, Kikuchi A, et al., 2003, Cell Sheet Engineering for Myocardial Tissue Reconstruction. Biomaterials, 24:2309–16. https://doi.org/10.1016/s0142-9612(03)00110-8
44. Dahl SL, Rhim C, Song YC, et al., 2007, Mechanical Properties and Compositions of Tissue Engineered and Native Arteries. Ann Biomed Eng, 35:348–55. https://doi.org/10.1007/s10439-006-9226-1
45. Yu Y, Haghiashtiani G, Hübscher T, et al., 2016, Three dimensional Bioprinting Using Self-assembling Scalable Scaffold-free “Tissue Strands” as a New Bioink. Sci Rep, 6:1–11.
46. Alblawi A, Ranjani AS, Yasmin H, et al., 2020, Scaffold free: A Developing Technique in Field of Tissue Engineering. Comput Methods Programs Biomed, 185:105148. https://doi.org/10.1016/j.cmpb.2019.105148
47. De Pieri A, Rochev Y, Zeugolis DI, 2021, Scaffold-free Cell based Tissue Engineering Therapies: Advances, Shortfalls and Forecast. NPJ Regen Med, 6:1–15.
48. Lee JM, Yeong WY, 2020, Engineering Macroscale Cell Alignment Through Coordinated Toolpath Design Using Support-assisted 3D Bioprinting. J R Soc Interface, 17:20200294.
49. Lee JM, Yeong WY, 2016, Design and Printing Strategies in 3D Bioprinting of Cell-hydrogels: A Review. Adv Healthc Mater, 5:2856–65. https://doi.org/10.1002/adhm.201600435
50. Lee JM, Ng WL, Yeong WY, 2019, Resolution and Shape in Bioprinting: Strategizing towards Complex Tissue and Organ Printing. Appl Phys Rev, 6:011307.
51. Prendergast ME, Davidson MD, Burdick JA, 2021, A Biofabrication Method to Align Cells within Bioprinted Photocrosslinkable and Cell-degradable Hydrogel Constructs Via Embedded Fibers. Biofabrication, 13:044108. https://doi.org/10.1088/1758-5090/ac25cc
52. Yue K, Santiago GT, Alvarez MM, et al., 2015, Synthesis, Properties, and Biomedical Applications of Gelatin Methacryloyl (GelMA) Hydrogels. Biomaterials, 73:254–71. https://doi.org/10.1016/j.biomaterials.2015.08.045
53. Ying G, Jiang N, Yu C, et al., 2018, Three-dimensional Bioprinting of Gelatin Methacryloyl (GelMA). Biodes Manuf, 1:215–24.
54. Ouyang L, Armstrong JP, Chen Q, et al., 2019, Void-free 3D Bioprinting for In Situ Endothelialization and Microfluidic Perfusion. Adv Funct Mater, 30:1908349. https://doi.org/10.1002/adfm.201908349
55. Muir VG, Burdick JA, 2020, Chemically Modified Biopolymers for the Formation of Biomedical Hydrogels. Chem Rev, 121:10908–49. https://doi.org/10.1021/acs.chemrev.0c00923
56. Burdick JA, Prestwich GD, 2011, Hyaluronic Acid Hydrogels for Biomedical Applications. Adv Mater, 23:H41–56. https://doi.org/10.1002/adma.201003963
57. Wade RJ, Bassin EJ, Gramlich WM, et al., 2015, Nanofibrous Hydrogels with Spatially Patterned Biochemical Signals to Control Cell Behavior. Adv Mater, 27: 1356–62. https://doi.org/10.1002/adma.201404993
58. Davidson MD, Ban E, Schoonen AC, et al., 2020, Mechanochemical Adhesion and Plasticity in Multifiber Hydrogel Networks. Adv Mater, 32:e1905719. https://doi.org/10.1002/adma.201905719
59. Gramlich WM, Kim IL, Burdick JA, 2013, Synthesis and Orthogonal Photopatterning of Hyaluronic Acid Hydrogels with Thiol-norbornene Chemistry. Biomaterials, 34:9803–11. https://doi.org/10.1016/j.biomaterials.2013.08.089
60. Mendes BB, Gómez-Florit M, Hamilton AG, et al., 2019, Human Platelet Lysate-Based Nanocomposite Bioink for Bioprinting Hierarchical Fibrillar Structures. Biofabrication, 12:015012. https://doi.org/10.1088/1758-5090/ab33e8
61. Munaz A, Vadivelu RK, St. John J, et al., 2016, Three dimensional Printing of Biological Matters. J Sci Adv Mater Dev, 1:1–17.
62. Cho DW, Kim BS, Jang J, et al., 2019, Various Applications of 3D-Bioprinted Tissues/Organs Using Tissue-Specific Bioinks. In: 3D Bioprinting. Berlin: Springer. p53–108.
63. Khoeini R, Nosrati H, Akbarzadeh A, et al., 2021, Natural and Synthetic Bioinks for 3D Bioprinting. Adv NanoBiomed Res, 1:2000097. https://doi.org/10.1002/anbr.202000097
64. Lee K, Cha C, 2020, Advanced Polymer-based Bioink Technology for Printing Soft Biomaterials. Macromol Res, 28:689–702.
65. Zarrintaj P, Manouchehri S, Ahmadi Z, et al., 2018, Agarosebased Biomaterials for Tissue Engineering. Carbohydr Polym, 187:66–84. https://doi.org/10.1016/j.carbpol.2018.01.060
66. Aydin L, Kucuk S, Kenar H, 2020, A Universal Selferoding Sacrificial Bioink that Enables Bioprinting at Room Temperature. Polym Adv Technol, 31:1634–47.
67. Kirchmajer DM, Gorkin R 3rd, 2015, An Overview of the Suitability of Hydrogel-Forming Polymers for Extrusion based 3D-printing. J Mater Chem B, 3:4105–17.
68. Alnoch RC, dos Santos LA, de Almeida JM, et al., 2020, Recent Trends in Biomaterials for Immobilization of Lipases for Application in Non-conventional Media. Catalysts, 10:697. https://doi.org/10.3390/catal10060697
69. Lee KY, Mooney DJ, 2012, Alginate: Properties and Biomedical Applications. Prog Polym Sci, 37:106–26. https://doi.org/10.1016/j.progpolymsci.2011.06.003
70. Chen Y, Xiong X, Liu X, et al., 2020, 3D Bioprinting of Shear-thinning Hybrid Bioinks with Excellent Bioactivity Derived from Gellan/Alginate and Thixotropic Magnesium Phosphate-based Gels. J Mater Chem B, 8:5500–14.
71. Axpe E, Oyen ML, 2016, Applications of Alginate-based Bioinks in 3D Bioprinting. Int J Mol Sci, 17:1976. https://doi.org/10.3390/ijms17121976
72. Piras CC, Smith DK, 2020, Multicomponent Polysaccharide Alginate-based Bioinks. J Mater Chem B, 8:8171–88.
73. Wang Q, Sun J, Yao Q, et al., 2018, 3D Printing with Cellulose Materials. Cellulose, 25:4275–301.
74. Nasatto PL, Pignon F, Silveira JL, et al., 2015, Methylcellulose, a Cellulose Derivative with Original Physical Properties and Extended Applications. Polymers, 7:777–803. https://doi.org/10.3390/polym7050777
75. Janarthanan G, Trana HN, Cha E, et al., 2020, 3D Printable and Injectable Lactoferrin-loaded Carboxymethyl Cellulose glycol Chitosan Hydrogels for Tissue Engineering Applications. Mater Sci Eng C, 113:111008. https://doi.org/10.1016/j.msec.2020.111008
76. Wu Y, Lin ZY, Wenger AC, et al., 2018, 3D Bioprinting of Liver-mimetic Construct with Alginate/Cellulose Nanocrystal Hybrid Bioink. Bioprinting, 9:1–6. https://doi.org/10.1016/j.bprint.2017.12.001
77. Jiang Y, Shi H, Zhao G, et al., 2020, Preparation of Cellulose Nanocrystal/Oxidized Dextran/Gelatin (CNC/OD/GEL) Hydrogels and Fabrication of a CNC/OD/GEL Scaffold by 3D Printing. J Mater Sci, 55:2618–35.
78. Markstedt K, Mantas A, Tournier I, et al., 2015, 3D Bioprinting Human Chondrocytes with Nanocellulose-Alginate Bioink for Cartilage Tissue Engineering Applications. Biomacromolecules, 16:1489–96.
79. Finny AS, Popoola O, Andreescu S, 2021, 3D-Printable Nanocellulose-based Functional Materials: Fundamentals and Applications. Nanomaterials (Basel), 11:2358. https://doi.org/10.3390/nano11092358
80. He Y, Derakhshanfar S, Zhong W, et al., 2020, Characterization and Application of Carboxymethyl Chitosan-Based Bioink in Cartilage Tissue Engineering. J Nanomater, 2020:2057097. https://doi.org/10.1155/2020/2057097
81. Wu Q, Therriault D, Heuzey MC, 2018, Processing and Properties of Chitosan Inks for 3D Printing of Hydrogel Microstructures. ACS Biomater Sci Eng, 4:2643–52. https://doi.org/10.1021/acsbiomaterials.8b00415
82. Yadav M, Goswami P, Paritosh K, et al., 2019, Seafood Waste: A Source for Preparation of Commercially Employable Chitin/Chitosan Materials. Bioresour Bioprocess, 6:1–20.
83. Shoulders MD, Raines RT, 2009, Collagen Structure and Stability. Annu Rev Biochem, 78:929–58. https://doi.org/10.1146/annurev.biochem.77.032207.120833
84. Guo K, Wang H, Li S, et al., 2021, Collagen-based Thiol- Norbornene Photoclick Bio-ink with Excellent Bioactivity and Printability. ACS Appl Mater Interfaces, 13:7037–50.
85. Keane TJ, Swinehart IT, Badylak SF, 2015, Methods of Tissue Decellularization Used for Preparation of Biologic Scaffolds and In Vivo Relevance. Methods, 84:25–34. https://doi.org/10.1016/j.ymeth.2015.03.005
86. Dzobo K, Motaung KSC, Adesida A, 2019, Recent Trends in Decellularized Extracellular Matrix Bioinks for 3D Printing: An Updated Review. Int J Mol Sci, 20:4628. https://doi.org/10.3390/ijms20184628
87. Vepari C, Kaplan DL, 2007, Silk as a Biomaterial. Prog Polym Sci, 32:991–1007. https://doi.org/10.1016/j.progpolymsci.2007.05.013
88. Kundu B, Kurland NE, Bano S, et al., 2014, Silk Proteins for Biomedical Applications: Bioengineering Perspectives. Prog Polym Sci, 39:251–67.
89. Rodriguez MJ, Brown J, Giordano J, et al., 2017, Silk Based Bioinks for Soft Tissue Reconstruction using 3-Dimensional (3D) Printing with In Vitro and In Vivo Assessment Biomaterials, 117:105–15. https://doi.org/10.1016/j.biomaterials.2016.11.046
90. Singh YP, Bandyopadhyay A, Mandal BB, 2019, 3D Bioprinting using Cross-Linker-Free Silk–Gelatin Bioink for Cartilage Tissue Engineering. ACS Appl Mater Interfaces, 11:33684–96.
91. Dingle YT, Bonzanni M, Liaudanskaya V, et al., 2021, Integrated Functional Neuronal Network Analysis of 3D Silk-Collagen Scaffold-Based Mouse Cortical Culture. STAR Protoc, 2:100292. https://doi.org/10.1016/j.xpro.2020.100292
92. Chawla S, Midha S, Sharma A, et al., 2018, Silk-based Bioinks for 3D Bioprinting. Adv Healthc Mater, 7:1701204. https://doi.org/10.1002/adhm.201701204
93. Chew KW, Show PL, Yap YJ, et al., 2018, Sonication and Grinding Pre-treatments on Gelidium Amansii Seaweed for the Extraction and Characterization of Agarose. Front Environ Sci Eng, 12:1–7.
94. Phanthong P, Reubroycharoen P, Hao X, et al., 2018, Nanocellulose: Extraction and Application. Carbon Resour Conv, 1:32–43. https://doi.org/10.1016/j.crcon.2018.05.004
95. Elieh-Ali-Komi D, Hamblin MR, 2016, Chitin and chitosan: Production and application of versatile biomedical nanomaterials. Int J Adv Res, 4:411.
96. Elieh-Ali-Komi D, Hamblin MR, 2016, Chitin and Chitosan: Production and Application of Versatile Biomedical Nanomaterials. Int J Adv Res (Indore), 4:411–27.
97. Kou SG, Peters LM, Mucalo MR, 2021, Chitosan: A Review of Sources and Preparation Methods. Int J Biol Macromol, 169:85–94. https://doi.org/10.1016/j.ijbiomac.2020.12.005
98. Sibilla S, Godfrey M, Brewer S, et al., 2015, An Overview of the Beneficial Effects of Hydrolysed Collagen as a Nutraceutical on Skin Properties: Scientific Background and Clinical Studies. Open Nutraceutical J, 8:29–42.
99. Patino MG, Neiders ME, Andreana S, et al., 2002, Collagen: An Overview. Implant Dent, 11:280–5. https://doi.org/10.1097/00008505-200207000-00014
100. Nimni ME, 1980, The Molecular Organization of Collagen and its Role in Determining the Biophysical Properties of the Connective Tissues. Biorheology, 17:51–82. https://doi.org/10.3233/bir-1980-171-210
101. Kim YS, Majid M, Melchiorri AJ, et al., 2019, Applications of Decellularized Extracellular Matrix in Bone and Cartilage Tissue Engineering. Bioeng Transl Med, 4:83–95.
102. Frantz C, Stewart KM, Weaver VM, 2010, The Extracellular Matrix at a Glance. J Cell Sci, 123:4195–200. https://doi.org/10.1242/jcs.023820
103. Sackett SD, Tremmel DM, Ma F, et al., 2018, Extracellular Matrix Scaffold and Hydrogel Derived from Decellularized and Delipidized Human Pancreas. Sci Rep, 8:10452. https://doi.org/10.1038/s41598-018-28857-1
104. Alipal J, Wang Y, Liu J, et al., 2021, A Review of Gelatin: Properties, Sources, Process, Applications, and Commercialisation. Mater Today Proc, 42:240–50.
105. Guvendiren M, Burdick JA, 2013, Engineering Synthetic Hydrogel Microenvironments to Instruct Stem Cells. Curr Opin Biotechnol, 24:841–6. https://doi.org/10.1016/j.copbio.2013.03.009
106. Billiet T, Vandenhaute M, Schelfhout J, et al., 2012, A Review of Trends and Limitations in Hydrogel-Rapid Prototyping for Tissue Engineering. Biomaterials, 33:6020–41. https://doi.org/10.1016/j.biomaterials.2012.04.050
107. Liu F, Zhou X, Cui F, et al., 2007, Synthesis and Properties of Poly (hydroxyethyl methacrylate) Hydrogel for IOL Materials. Sheng Wu Yi Xue Gong Cheng Xue Za Zhi, 24:595–8.
108. Yan Y, Xiong Z, Hu Y, et al., 2003, Layered Manufacturing of Tissue Engineering Scaffolds Via Multi-Nozzle Deposition. Mater Lett, 57:2623–8.
109. Guedes AC, Amaro HM, Malcata FX, 2011, Microalgae as Sources of High Added-Value Compounds a Brief Review of Recent Work. Biotechnol Prog, 27:597–613. https://doi.org/10.1002/btpr.575
110. Moreno-Garrido I, 2008, Microalgae Immobilization: Current Techniques and Uses. Bioresou Technol, 99:3949–64. https://doi.org/10.1016/j.biortech.2007.05.040
111. De-Bashan LE, Bashan Y, 2010, Immobilized Microalgae for Removing Pollutants: Review of Practical Aspects. Bioresour Technol, 101:1611–27. https://doi.org/10.1016/j.biortech.2009.09.043
112. Ferro Y, Perullini M, Jobbagy M, et al., 2012, Development of a Biosensor for Environmental Monitoring Based on Microalgae Immobilized In Silica Hydrogels. Sensors (Basel), 12:16879–91. https://doi.org/10.3390/s121216879
113. Malik S, Hagopian J, Mohite S, et al., 2020, Robotic Extrusion of Algae-laden Hydrogels for Large-scale Applications. Glob Chall, 4:1900064. https://doi.org/10.1002/gch2.201900064
114. Zhao S, Guo C, Kumarasena A, et al., 2019, 3D Printing of Functional Microalgal Silk Structures for Environmental Applications. ACS Biomater Sci Eng, 5:4808–16. https://doi.org/10.1021/acsbiomaterials.9b00554
115. Zheng Z, Wu J, Liu M, et al., 2018, 3D Bioprinting of Self standing Silk-based Bioink. Adv Healthc Mater, 7:1701026. https://doi.org/10.1002/adhm.201701026
116. Thakare K, Jerpseth L, Qin H, et al., 2020, Bioprinting Using Algae: Effects of Extrusion Pressure and Needle Diameter on Cell Quantity in Printed Samples. J Manuf Sci Eng, 143:014501. https://doi.org/10.1115/1.4048853
117. Russo R, Abbate M, Malinconico M, et al., 2010, Effect of Polyglycerol and the Crosslinking on the Physical Properties of a Blend Alginate-Hydroxyethylcellulose. Carbohydr Polym, 82:1061–7. https://doi.org/10.1016/j.carbpol.2010.06.037
118. Balasubramanian S, Yu K, Meyer AS, et al., 2021, Bioprinting of Regenerative Photosynthetic Living Materials. Adv Funct Mater, 31:2011162. https://doi.org/10.1002/adfm.202011162
119. Kumar V, Vlaskin MS, Grigorenko AV, 2021, 3D Bioprinting to Fabricate Living Microalgal Materials. Trends Biotechnol, 39:1243–4. https://doi.org/10.1016/j.tibtech.2021.10.006
120. Santmarti A, Zhang H, Lappalainen T, et al., 2020, Cellulose Nanocomposites Reinforced with Bacterial Cellulose Sheets Prepared from Pristine and Disintegrated Pellicle. Compos Part A Appl Sci Manuf, 130:105766. https://doi.org/10.1016/j.compositesa.2020.105766
121. Rahman MM, Netravali AN, 2016, Aligned Bacterial Cellulose Arrays as “Green” Nanofibers for Composite Materials. ACS Macro Lett, 5:1070–4. https://doi.org/10.1021/acsmacrolett.6b00621
122. Yu K, Balasubramanian S, Pahlavani H, et al., 2020, Spiral Honeycomb Microstructured Bacterial Cellulose for Increased Strength and Toughness. ACS Appl Mater Interfaces, 12:50748–55. https://doi.org/10.1021/acsami.0c15886
123. Wang S, Li T, Chen C, et al., 2018, Transparent, Anisotropic Biofilm with Aligned Bacterial Cellulose Nanofibers. Adv Funct Mater, 28:1707491. https://doi.org/10.1002/adfm.201707491
124. Florea M, Hagemann H, Santosa G, et al., 2016, Engineering Control of Bacterial Cellulose Production Using a Genetic Toolkit and a New Cellulose-Producing Strain. Proc Natl Acad Sci, 113:E3431–40. https://doi.org/10.1073/pnas.1522985113
125. Thakare K, Jerpseth L, Pei Z, et al., 2021, Three-dimensional Printing of Hydrogel Filters Containing Algae Cells for Copper Removal from Contaminated Water. J Manuf Sci Eng, 143:104502. https://doi.org/10.1115/1.4050761
126. Bajpai S, Sharma S, 2004, Investigation of Swelling/Degradation Behaviour of Alginate Beads Crosslinked with Ca2+ and Ba2+ ions. React Funct Polym, 59:129–40. https://doi.org/10.1016/j.reactfunctpolym.2004.01.002
127. Malik KA, 1995, A Convenient Method to Maintain Unicellular Green Algae for Long Times as Standing Liquid Cultures. J Microbiol Methods, 22:221–7. https://doi.org/10.1016/0167-7012(95)00005-6 128. Reynolds CS, 2006, The Ecology of Phytoplankton. Cambridge, United Kingdom: Cambridge University Press.
128. Reynolds CS, 2006, The Ecology of Phytoplankton. Cambridge, United Kingdom: Cambridge University Press.
129. Co JR, Culaba AB, 2019, 3D Printing: Challenges and Opportunities of an Emerging Disruptive Technology. In: 2019 IEEE 11th International Conference on Humanoid, Nanotechnology, Information Technology, Communication and Control, Environment, and Management (HNICEM), IEEE.
130. Poomathi N, Singh S, Prakash C, et al., 2020, 3D Printing in Tissue Engineering: A State of the Art Review of Technologies and Biomaterials. Rapid Prototyp J, 26:1313–34. https://doi.org/10.1108/RPJ-08-2018-0217
131. Stefanova A, In-Na P, Caldwell GS, et al., 2021, Photosynthetic Textile Biocomposites: Using Laboratory Testing and Digital Fabrication to Develop Flexible Living Building Materials. Sci Eng Compos Mater, 28:223–36. https://doi.org/10.1515/secm-2021-0023
132. McHugh DJ, 2003, A Guide to the Seaweed Industry. Vol. 441. United Nations, Rome: Food and Agriculture Organization.
133. Shahidi F, Synowiecki J, 1991, Isolation and Characterization of Nutrients and Value-added Products from Snow Crab (Chionoecetes opilio) and Shrimp (Pandalus borealis) Processing Discards. J Agric Food Chem, 39:1527–32.
134. Surjushe A, Vasani R, Saple D, 2008, Aloe vera: A Short Review. Indian J Dermatol, 53:163–6. https://doi.org/10.4103/0019-5154.44785
135. Calvert P, 2016, 3D Printing of Gels with Living Photosynthetic Algae. MRS Adv, 1:2569–72.
135. Calvert P, 2016, 3D Printing of Gels with Living Photosynthetic Algae. MRS Adv, 1:2569–72.
136. Oey ML, Vanstreels E, Baerdemaeker JD, et al., 2007, Effect of Turgor on Micromechanical and Structural Properties of Apple Tissue: A Quantitative Analysis. Postharvest Biology and Technology, 44:240–7.
137. Nilsson SB, Hertz CH, Falk S, 1958, On the Relation Between Turgor Pressure and Tissue Rigidit Y. II: Theoretical Calculations on Model Systems. Physiol Plant, 11:818–37.
138. Tyree M, Jarvis P, 1982, Water in tissues and cells. In: Physiological Plant Ecology II. Berlin, Germany: Springer p35–77.
139. Sila DN, Buggenhout V, Duvetter T, et al., 2009, Pectins in Processed Fruits and Vegetables: Part II-structure-function Relationships. Compr Rev Food Sci Food Saf, 8:86–104. https://doi.org/10.1111/j.1541-4337.2009.00071.x
140. Van Buren J, 1991, Function of Pectin in Plant Tissue Structure and Firmness. The Chemistry and Technology of Pectin. New York: Academic Press. p1–22.
141. Vancauwenberghe V, et al., 2019, 3D Printing of Plant Tissue for Innovative Food Manufacturing: Encapsulation of Alive Plant Cells into Pectin Based Bio-ink. J Food Eng, 263:454–64. https://doi.org/10.1016/J.JFOODENG.2017.12.003
142. Willats WG, Orfila C, Limberg G, et al., 2001, Modulation of the Degree and Pattern of Methyl-esterification of Pectic Homogalacturonan in Plant Cell Walls: Implications for Pectin Methyl Esterase Action, Matrix Properties, and Cell Adhesion. J Biol Chem, 276:19404–13. https://doi.org/10.1074/jbc.M011242200
143. Vancauwenberghe V, Katalagarianakis L, Wang Z, et al., 2017, Pectin Based Food-ink Formulations for 3-D Printing of Customizable Porous Food Simulants. Innov Food Sci Emerg Technol, 42:138–150. https://doi.org/10.1016/j.ifset.2017.06.011
144. Fraeye I, Duvetter T, Doungla E, et al., 2010, Fine-tuning the Properties of Pectin–Calcium Gels by Control of Pectin Fine Structure, Gel Composition and Environmental Conditions. Trends Food Sci Technol, 21:219–28. https://doi.org/10.1016/j.tifs.2010.02.001
145. Fu J, Rao M, 2001, Rheology and Structure Development During Gelation of Low-Methoxyl Pectin Gels: The Effect of Sucrose. Food Hydrocoll, 15:93–100. https://doi.org/10.1016/S0268-005X(00)00056-4
146. Mbong VBM, Ampofo-Asiama J, Hertog ML, et al., 2017, The Effect of Temperature on the Metabolic Response of Lamb’s Lettuce (Valerianella locusta, (L), Laterr.) Cells to Sugar Starvation. Postharvest Biol Technol, 125:1–12. https://doi.org/10.1016/j.postharvbio.2016.10.013
147. Bohren CF, Huffman DR, 2008, Absorption and Scattering of Light by Small Particles. Hoboken, New Jersey: John Wiley and Sons.
148. Chen L, Tang X, Xie P, et al., 2018, 3D Printing of Artificial Leaf with Tunable Hierarchical Porosity for CO2 Photoreduction. Chem Mater, 30:799–806. https://doi.org/10.1021/acs.chemmater.7b04313
149. Arango MA, Kwakye-Ackah D, Agarwal S, et al., 2017, Environmentally Friendly Engineering and Three dimensional Printing of TiO2 Hierarchical Mesoporous Cellular Architectures. ACS Sustain Chem Eng, 5:10421–9. https://doi.org/10.1021/acssuschemeng.7b02450
150. Zhou H, Guo J, Li P, et al., 2013, Leaf-architectured 3D Hierarchical Artificial Photosynthetic System of Perovskite Titanates towards CO2 Photoreduction into Hydrocarbon Fuels. Sci Rep, 3:1667. https://doi.org/10.1038/srep01667
151. Gratson GM, Lewis JA, 2005, Phase Behavior and Rheological Properties of Polyelectrolyte Inks for Directwrite Assembly. Langmuir, 21:457–64. https://doi.org/10.1021/la048228d
152. Smay JE, Gratson GM, Shepherd RF, et al., 2002, Directed Colloidal Assembly of 3D Periodic Structures. Adv Mater, 14:1279–83. https://doi.org/10.1002/1521-4095(20020916)14:18%3C1279: AID-ADMA1279%3E3.0.CO;2-A
153. Zhu C, Smay JE, 2011, Thixotropic Rheology of Concentrated Alumina Colloidal Gels for Solid Freeform Fabrication. J Rheol, 55:655–72. https://doi.org/10.1122/1.3573828
154. Matter PH, Wang E, Ozkan US, 2006, Preparation of Nanostructured Nitrogen-Containing Carbon Catalysts for the Oxygen Reduction Reaction from SiO2-and MgO-Supported Metal Particles. J Catal, 243:395–403. https://doi.org/10.1016/j.jcat.2006.07.029
155. Varma A, Gemeda HB, McNulty MJ, et al., 2021, Immobilization of Transgenic Plant Cells towards Bioprinting for Production of a Recombinant Biodefense Agent. Biotechnol J, 16:2100133. https://doi.org/10.1002/biot.202100133
156. Corbin JM, Hashimoto BI, Karuppanan K, et al., 2016, Semicontinuous Bioreactor Production of Recombinant Butyrylcholinesterase in Transgenic Rice Cell Suspension Cultures. Front Plant Sci, 7:412. https://doi.org/10.3389/fpls.2016.00412
157. Macharoen K, McDonald KA, Nandi S, 2020, Simplified Bioreactor Processes for Recombinant Butyrylcholinesterase Production in Transgenic Rice Cell Suspension Cultures. Biochem Eng J, 163:107751. https://doi.org/10.1016/j.bej.2020.107751
158. Schillberg S, Raven N, Spiegel H, et al., 2019, Critical Analysis of the Commercial Potential of Plants for the Production of Recombinant Proteins. Front Plant Sci, 10:720. https://doi.org/10.3389/fpls.2019.00720
159. Wangpraseurt D, You S, Azam F, et al., 2020, Bionic 3D Printed Corals. Nat Commun, 11:1–8. https://doi.org/10.1038/s41467-020-15486-4
160. Wangpraseurt D, Jacques SL, Petrie T, et al., 2016, Monte Carlo Modeling Of Photon Propagation Reveals Highly Scattering Coral Tissue. Front Plant Sci, 7:1404. https://doi.org/10.3389/fpls.2016.01404
161. Roth MS, 2014, The Engine of the Reef: Photobiology of the Coral-Algal Symbiosis. Front Microbiol, 5:422. https://doi.org/10.3389/fmicb.2014.00422
162. Brodersen KE, Lichtenberg M, Ralph PJ, et al., 2014, Radiative Energy Budget Reveals High Photosynthetic Efficiency in Symbiont-Bearing Corals. J R Soc Interface, 11:20130997. https://doi.org/10.1098/rsif.2013.0997
163. Enríquez S, Méndez ER, Hoegh-Guldberg O, et al., 2017, Key Functional Role of the Optical Properties of Coral Skeletons in Coral Ecology and Evolution. Proc R Soc B Biol Sci, 284:1667. https://doi.org/10.1098/rspb.2016.1667
164. Murphy SV, Atala A, 2014, 3D Bioprinting of Tissues and Organs. Nat Biotechnol, 32:773–85. https://doi.org/10.1038/nbt.2958
165. Liu L, Choi S, 2019, A 3D Printed Cyanobacterial Leaf for Carbon Dioxide Reduction. in 2019 IEEE 32nd International Conference on Micro Electro Mechanical Systems (MEMS), IEEE.
166. Liu L, Choi S, 2017, Self-sustainable, High-power-density Bio-solar Cells For Lab-on-a-Chip Applications. Lab Chip, 17:3817–25.
167. De la Vega L, Gómez DA, Abelseth E, et al., 2018, 3D Bioprinting Human Induced Pluripotent Stem Cell-derived Neural Tissues Using a Novel Lab-on-a-Printer Technology. Appl Sci, 8:2414. https://doi.org/10.3390/app8122414