The effect of 3D-printed bone tissue scaffolds geometrical designs on bacterial biofilm formation
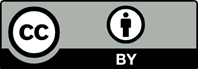
Bone fractures are recognized as a global health problem. A common strategy to tackle this issue is to employ a tissue engineering scaffold to accelerate tissue healing. However, one of the main challenges that can result in delaying the recovery is the risk of bacterial infections. This study aims to assess the impact of the geometry and the porosity of tissue scaffolds on the Staphylococcus aureus biofilm formation. Three triply periodic minimal surface designs of Schwarz primitive (SP), gyroid (GY), and Schwarz diamond (SD) and re-entrant auxetic (RE) design were examined and compared to a reference design (RD) considering two different porosity levels of 75% and 45%. The amount of biofilm was quantified using crystal violet assay and was visualized using scanning electron microscopy. The SP scaffold, with low porosity, exhibited a significantly less amount of bacterial biofilm formation and was regarded as having the best design among the others, while the SD with low porosity showed the greatest amount of biofilm. The morphological analysis was also in line with the crystal violet assay results. On the other hand, the surface roughness was affected by the complexity, geometrical variations, and limitations of fused filament fabrication three-dimensional printing. For the RD, SP, GY, and SD designs, an increase in surface roughness was demonstrated to increase the production of bacterial biofilms. Without statistical significance, the RE design showed the opposite trend. Contrary to other designs, the increase in pore size of the SP and GY designs was associated with the development of bacterial biofilms. This study suggests that it is possible to minimize the likelihood of bacterial biofilm formation by optimizing the scaffold geometry and its manufacturing.
- Al-Tamimi A, Fernandes PRA, Peach C, et al. Metallic bone fixation implants: A novel design approach for reducing the stress shielding phenomenon. Virtual Phys Prototyp. 2017;12(2):141-151. doi: 10.1080/17452759.2017.1307769
- Murphy CM, O’Brien FJ. Understanding the effect of mean pore size on cell activity in collagen-glycosaminoglycan scaffolds. Cell Adh Migr. 2010;4(3):377-381. doi: 10.4161/cam.4.3.11747
- iHealthcareAnalyst I. Global bone fracture repair devices market $22.3 billion by 2029. November 27, 2023. https://www.ihealthcareanalyst.com/global-bone-fracture-repair-devices-market/
- Hutmacher DW, Tandon B, Dalton PD. Scaffold design and fabrication. In: De Boer J, Blitterswijk CAV, Uquillas JA, Malik N, eds. Tissue Engineering (Third Edition). Academic Press; Cambridge, Massachusetts, USA. 2023:355-385.
- Zadpoor AA. Meta-biomaterials. Biomater Sci. 2020;8(1):18-38. doi: 10.1039/C9BM01247H
- Deng F, Liu L, Li Z, Liu J. 3D printed Ti6Al4V bone scaffolds with different pore structure effects on bone ingrowth. J Bio Eng. 2021;15(1):4. doi: 10.1186/s13036-021-00255-8
- Qin Y, Wang Q, Shi C, et al. Structural design and performance study of primitive triply periodic minimal surfaces Ti6Al4V biomimetic scaffold. Sci Rep. 2022;12(1):12759. doi: 10.1038/s41598-022-17066-6
- Asbai-Ghoudan R, Ruiz de Galarreta S, Rodriguez-Florez N. Analytical model for the prediction of permeability of triply periodic minimal surfaces. J Mech Behav Biomed Mater. 2021;124:104804. doi: 10.1016/j.jmbbm.2021.104804
- Lvov VA, Senatov FS, Veveris AA, Skrybykina VA, Díaz Lantada A. Auxetic metamaterials for biomedical devices: current situation, main challenges, and research trends. Materials (Basel). 2022;15(4):1439.
- Mardling P, Alderson A, Jordan-Mahy N, Le Maitre CL. The use of auxetic materials in tissue engineering. Biomater Sci. 2020;8(8):2074-2083. doi: 10.1039/C9BM01928F
- Choi HJ, Lee JJ, Park YJ, et al. MG-63 osteoblast-like cell proliferation on auxetic PLGA scaffold with mechanical stimulation for bone tissue regeneration. Biomater Res. 2016;20:33. doi: 10.1186/s40824-016-0080-4
- Park YJ, Kim JK. The effect of negative Poisson’s ratio polyurethane scaffolds for articular cartilage tissue engineering applications. Adv Mater Sci Eng. 2013;2013:853289. doi: 10.1155/2013/853289
- Rahmani R, Antonov M, Kollo L, Holovenko Y, Prashanth KG. Mechanical behavior of Ti6Al4V scaffolds filled with CaSiO3 for implant applications. App Sci. 2019;9(18):3844. doi: 10.3390/app9183844
- Callens SJP, Uyttendaele RJC, Fratila-Apachitei LE, Zadpoor AA. Substrate curvature as a cue to guide spatiotemporal cell and tissue organization. Biomater. 2020;232:119739. doi: 10.1016/j.biomaterials.2019.119739
- Zheng X, Guo X, Watanabe I. A mathematically defined 3D auxetic metamaterial with tunable mechanical and conduction properties. Mater Des. 2021;198:109313. doi: 10.1016/j.matdes.2020.109313
- Veerabagu U, Palza H, Quero F. Review: Auxetic polymer-based mechanical metamaterials for biomedical applications. ACS Biomater Sci Eng. 2022;8(7):2798-2824. doi: 10.1021/acsbiomaterials.2c00109
- Cheng A, Schwartz Z, Kahn A, et al. Advances in porous scaffold design for bone and cartilage tissue engineering and regeneration. Tissue Eng Part B Rev. 2018;25(1):14-29. doi: 10.1089/ten.teb.2018.0119
- Eltom A, Zhong G, Muhammad A. Scaffold techniques and designs in tissue engineering functions and purposes: A review. Adv Mater Sci Eng. 2019;2019:3429527. doi: 10.1155/2019/3429527
- Milovanović J, Stojković M, Trifunović M, Vitković N. Review of bone scaffold design concepts and design methods. Facta Universitatis, Series: Mechanical Engineering. 2023;21(1):151-173. doi: 1022190/FUME200328038M.04/10/2023
- Liu F, Mishbak H, Bartolo P. Hybrid polycaprolactone/ hydrogel scaffold fabrication and in-process plasma treatment using PABS. Int J Bioprint. 2019;5(1):174. doi: 10.18063/ijb.v5i1.174
- Huang B, Caetano G, Vyas C, et al. Polymer-ceramic composite scaffolds: The effect of hydroxyapatite and β-tri-calcium phosphate. Materials (Basel). 2018;11(1):129-142. doi: 10.3390/ma11010129
- Bayart M, Charlon S, Soulestin J. Fused filament fabrication of scaffolds for tissue engineering; how realistic is shape-memory? A review. Polymers. 2021;217:123440. doi: 10.1016/j.polymer.2021.123440
- Biswal T. Biopolymers for tissue engineering applications: A review. Mater Tod Proc. 2021;41:397-402. doi: 10.1016/j.matpr.2020.09.628
- Veeman D, Sai MS, Sureshkumar P, et al. Additive manufacturing of biopolymers for tissue engineering and regenerative medicine: An overview, potential applications, advancements, and trends. Int J Polymer Sci. 2021;2021:4907027. doi: 10.1155/2021/4907027
- Gleadall A, Visscher D, Yang J, Thomas D, Segal J. Review of additive manufactured tissue engineering scaffolds: Relationship between geometry and performance. Burns Trauma. 2018;6(1):19. doi: 10.1186/s41038-018-0121-4
- O’Brien FJ. Biomaterials & scaffolds for tissue engineering. Mater Today. 2011;14(3):88-95. doi: 10.1016/S1369-7021(11)70058-X
- Zhao C, Liu W, Zhu M, Wu C, Zhu Y. Bioceramic-based scaffolds with antibacterial function for bone tissue engineering: A review. Bioact Mater. 2022;18:383-398. doi: 10.1016/j.bioactmat.2022.02.010
- Kostakioti M, Hadjifrangiskou M, Hultgren SJ. Bacterial biofilms: Development, dispersal, and therapeutic strategies in the dawn of the postantibiotic era. Cold Spring Harb Perspect Med. 2013;3(4):a010306. doi: 10.1101/cshperspect.a010306
- Yuan J, Wang B, Han C, et al. Nanosized-Ag-doped porous β-tricalcium phosphate for biological applications. Mater Sci Eng C Mater Biol Appl. 2020;114:111037. doi: 10.1016/j.msec.2020.111037
- Zhu T, Zhu M, Zhu Y. Fabrication of forsterite scaffolds with photothermal-induced antibacterial activity by 3D printing and polymer-derived ceramics strategy. Ceram Int. 2020;46(9):13607-13614. doi: 10.1016/j.ceramint.2020.02.146
- Chen Z, Xie YM, Wu X, et al. On hybrid cellular materials based on triply periodic minimal surfaces with extreme mechanical properties. Mater Des. 2019;183:108109. doi: 10.1016/j.matdes.2019.108109
- McCreight C. nTopology: What equations are used to create the TPMS types? Accessed August 19, 2023. https://support.ntop.com/hc/en-us/articles/360053267814- What-equations-are-used-to-create-the-TPMS-types-
- Ren X, Das R, Tran P, Ngo TD, Xie YM. Auxetic metamaterials and structures: A review. Smart Mater Struct. 2018;27(2):023001. doi: 10.1088/1361-665X/aaa61c
- Warner JJ, Gillies AR, Hwang HH, et al. 3D-printed biomaterials with regional auxetic properties. J Mech Behav Biomed Mater. 2017;76:145-152. doi: 10.1016/j.jmbbm.2017.05.016
- Kapnisi M, Mansfield C, Marijon C, et al. Auxetic cardiac patches with tunable mechanical and conductive properties toward treating myocardial infarction. Adv Funct Mater. 2018;28(21):1800618. doi: 10.1002/adfm.201800618
- Naveed N. Investigating the material properties and microstructural changes of fused filament fabricated PLA and tough-PLA parts. Polymers. 2021;13(9):1487. doi: 10.3390/polym13091487
- Priya G, Anitha R, Akila R, Narendra Kumar U, Manjubala I. Biofilm formation by S. aureus on composite scaffolds – A qualitative and quantitative in vitro analysis. Mater Today Proc. 2019;15:217-223. doi: 10.1016/j.matpr.2019.04.193
- Aldarhami A, Bazaid AS, Qanash H, et al. Effects of repeated in-vitro exposure to Saudi honey on bacterial resistance to antibiotics and biofilm formation. Infect Drug Resist. 2023;16:4273-4283. doi: 10.2147/idr.S410159
- Petrachi T, Resca E, Piccinno MS, et al. An alternative approach to investigate biofilm in medical devices: A feasibility study. Int J Environ Res Public Health. 2017;14(12):1587. doi: 10.3390/ijerph14121587
- Amin Yavari S, Croes M, Akhavan B, et al. Layer by layer coating for bio-functionalization of additively manufactured meta-biomaterials. Additiv Manuf. 2020;32:100991. doi: 10.1016/j.addma.2019.100991
- Poltue T, Karuna C, Khrueaduangkham S, Seehanam S, Promoppatum P. Design exploration of 3D-printed triply periodic minimal surface scaffolds for bone implants. Int J Mech Sci. 2021;211:106762.doi: 10.1016/j.ijmecsci.2021.106762
- Ali D. Effect of scaffold architecture on cell seeding efficiency: A discrete phase model CFD analysis. Comput Biol Med. 2019;109:62-69. doi: 10.1016/j.compbiomed.2019.04.025
- Kim Y, Son KH, Lee JW. Auxetic structures for tissue engineering scaffolds and biomedical devices. Materials (Basel). 2021;14(22). doi: 10.3390/ma14226821
- Hemmatian T, Lee H, Kim J. Bacteria adhesion of textiles influenced by wettability and pore characteristics of fibrous substrates. Polymers. 2021;13(2):223. doi: 10.3390/polym13020223
- Torres-Sanchez C, Al Mushref FRA, Norrito M, et al. The effect of pore size and porosity on mechanical properties and biological response of porous titanium scaffolds. Mater Sci Eng C Mater Biol Appl. 2017;77:219-228. doi: 10.1016/j.msec.2017.03.249
- Serrano-Aroca Á, Cano-Vicent A, Sabater i Serra R, et al. Scaffolds in the microbial resistant era: Fabrication, materials, properties and tissue engineering applications. Mater Today Bio. 2022;16:100412. doi: 10.1016/j.mtbio.2022.100412
- Golhin AP, Tonello R, Frisvad JR, Grammatikos S, Strandlie A. Surface roughness of as-printed polymers: A comprehensive review. Int J Adv Manuf Tech. 2023;127(3):987-1043. doi: 10.1007/s00170-023-11566-z
- Dezaki ML, Ariffin MKAM, Serjouei A, et al. Influence of infill patterns generated by CAD and FDM 3D printer on surface roughness and tensile strength properties. App Sci. 2021;11(16):7272.
- Zheng S, Bawazir M, Dhall A, et al. Implication of surface properties, bacterial motility, and hydrodynamic conditions on bacterial surface sensing and their initial adhesion. Front Bioeng Biotechnol. 2021;9:643722. doi: 10.3389/fbioe.2021.643722
- Dong Z, Zhao X. Application of TPMS structure in bone regeneration. Eng Reg. 2021;2:154-162. doi: 10.1016/j.engreg.2021.09.004
- Abbasi N, Hamlet S, Love RM, Nguyen N-T. Porous scaffolds for bone regeneration. J Sci Adv Mater Devices. 2020;5(1):1-9. doi: 10.1016/j.jsamd.2020.01.007
- Nam JH, Lee SY, Khan G, Park ES. Validation of the optimal scaffold pore size of nasal implants using the 3-dimensional culture technique. Arch Plast Surg. 2020;47(4):310-316. doi: 10.5999/aps.2020.00213
- Yao Y-t, Yang Y, Ye Q, et al. Effects of pore size and porosity on cytocompatibility and osteogenic differentiation of porous titanium. J Mater Sci Mater Med. 2021;32(6):72. doi: 10.1007/s10856-021-06548-0
- Zhang J, Chen X, Sun Y, et al. Design of a biomimetic graded TPMS scaffold with quantitatively adjustable pore size. Mater Des. 2022;218:110665. doi: 10.1016/j.matdes.2022.110665