3D-printed triaxial nozzles fabricated by stereolithography to prevent backflow in soft matter biofabrication
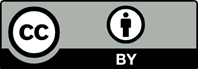
Three-dimensional (3D) bioprinting has a significant influence on tissue engineering by virtue of its capacity to produce complicated structures with complex geometries that are challenging to recreate using conventional manufacturing methods. However, the nozzle design and fabrication remain a limitation within extrusion-based 3D bioprinting, restricting and compromising the overall potential of this technology. The proposed nozzle design combines three Luer-Lok compatible inlets and an outlet within the printed body, eliminating manual assembly and enhancing fabrication consistency and quality. Furthermore, a finite element analysis of the fluid flow in the nozzle demonstrated the effectiveness of the nozzle to minimize backflow, in comparison with a traditional nozzle design. The tetrameric IIZK (Ac-Ile-IIe-Cha-Lys-NH2) and IIFK (Ac-Ile-IIe-Phe-Lys-NH2) peptide bioinks were used to 3D-print a variety of 3D scaffolds of varying complexity, with good resolution and gel continuity. Our work successfully demonstrated the fabrication of a novel design and its potential, and by means of 3D bioprinting, we assessed the biocompatibility and cell viability of the cell-laden constructs. This study highlights the capability of the novel design, which aids the field of tissue engineering, allowing 3D extrusion-based bioprinting to be utilized in the production of cell-incorporated constructions or scaffolds.
Charlotte A. E. Hauser serves as the Editorial Board Member of the journal, but did not in any way involve in the editorial and peer-review process conducted for this paper, directly or indirectly.
- Kafle A, Luis E, Silwal R, et al., 2021, 3D/4D printing of polymers: Fused deposition modelling (FDM), selective laser sintering (SLS), and stereolithography (SLA). Polymers (Basel), 13: 3101. https://doi.org/10.3390/polym13183101
- Mirzaali MJ, Moosabeiki V, Rajaai SM, et al., 2022, Additive manufacturing of biomaterials-design principles and their implementation. Materials (Basel), 15: 5457. https://doi.org/10.3390/ma15155457
- Mehrpouya M, Dehghanghadikolaei A, Fotovvati B, et al., 2019, The potential of additive manufacturing in the smart factory industrial 4.0: A review. Appl Sci, 9: 3865. https://doi.org/10.3390/app9183865
- Prashar G, Vasudev H, Bhuddhi D, 2022, Additive manufacturing: Expanding 3D printing horizon in industry 4.0. Int J Interact Des Manuf (IJIDeM), 6:1–15. https://doi.org/10.1007/s12008-022-00956-4
- Jiang Z, Diggle B, Tan ML, et al., 2020, Extrusion 3D printing of polymeric materials with advanced properties. Adv Sci (Weinh), 7: 2001379. https://doi.org/10.1002/advs.202001379
- Ligon SC, Liska R, Stampfl J, et al., 2017, Polymers for 3D printing and customized additive manufacturing. Chem Rev, 117: 10212–10290. https://doi.org/10.1021/acs.chemrev.7b00074
- Lin Q, Li L, Luo S, 2019, Asymmetric electrochemical catalysis. Chemistry, 25: 10033–10044. https://doi.org/10.1002/chem.201901284
- Zhang YS, Khademhosseini A, 2017, Advances in engineering hydrogels. Science, 356: eaaf3627. https://doi.org/10.1126/science.aaf3627
- Malda J, Visser J, Melchels FP, et al., 2013, 25th anniversary article: Engineering hydrogels for biofabrication. Adv Mater, 25: 5011–5028. https://doi.org/10.1002/adma.201302042
- Zhao F, Shi Y, Pan L, et al., 2017, Multifunctional nanostructured conductive polymer gels: Synthesis, properties, and applications. Acc Chem Res, 50: 1734–1743. https://doi.org/10.1021/acs.accounts.7b00191
- Ouyang L, Highley CB, Rodell CB, et al., 2016, 3D printing of shear-thinning hyaluronic acid hydrogels with secondary cross-linking. ACS Biomater Sci Eng, 2: 1743–1751. https://doi.org/10.1021/acsbiomaterials.6b00158
- Jungst T, Smolan W, Schacht K, et al., 2016, Strategies and molecular design criteria for 3D printable hydrogels. Chem Rev, 116: 1496–1539. https://doi.org/10.1021/acs.chemrev.5b00303
- Ouyang L, Highley CB, Sun W, et al., 2017, A generalizable strategy for the 3D bioprinting of hydrogels from nonviscous photo‐crosslinkable inks. Adv Mater, 29: 1604983. https://doi.org/10.1002/adma.201604983
- Wang Y, Zhang S, Wang J, 2021, Photo-crosslinkable hydrogel and its biological applications. Chin Chem Lett, 32: 1603–1614. https://doi.org/10.1016/j.cclet.2020.11.073
- Naghieh S, 2020, Extrusion Bioprinting of Hydrogel Scaffolds: Printability and Mechanical Behavior. Canada: University of Saskatchewan.
- Hauser CA, Deng R, Mishra A, et al., 2011, Natural tri- to hexapeptides self-assemble in water to amyloid beta-type fiber aggregates by unexpected alpha-helical intermediate structures. Proc Natl Acad Sci U S A, 108: 1361–1366. https://doi.org/10.1073/pnas.1014796108
- Loo Y, Chan YS, Szczerbinska I, et al., 2019, A chemically well-defined, self-assembling 3D substrate for long-term culture of human pluripotent stem cells. ACS Appl Bio Mater, 2: 1406–1412. https://doi.org/10.1021/acsabm.8b00686
- Mishra A, Loo Y, Deng R, et al., 2011, Ultrasmall natural peptides self-assemble to strong temperature-resistant helical fibers in scaffolds suitable for tissue engineering. Nano Today, 6: 232–239. https://doi.org/10.1016/j.nantod.2011.05.001
- Wu EC, Zhang S, Hauser CA, 2012, Self-assembling peptides as cell-interactive scaffolds. Adv Funct Mater, 22: 456–468. https://doi.org/10.1002/adfm.201101905
- Kahin K, Khan Z, Albagami M, et al., 2019, Development of a Robotic 3D Bioprinting and Microfluidic Pumping System for Tissue and Organ Engineering. In: Proceedings of SPIE, Microfluidics, BioMEMS, and Medical Microsystems XVII, San Francisco, United States.
- Khan Z, Kahin K, Rauf S, et al., 2019, Optimization of a 3D bioprinting process using ultrashort peptide bioinks. Int J Bioprinting, 5: 173. https://doi.org/10.18063/ijb.v5i1.173
- Khan Z, Kahin K, Hauser C, 2021, Time-dependent Pulsing of Microfluidic Pumps to Enhance 3D Bioprinting of Peptide Bioinks. In: Microfluidics, BioMEMS, and Medical Microsystems XIX, United States. https://doi.org/10.1117/12.2578830
- Abdelrahman S, Alsanie WF, Khan ZN, et al., 2022, A Parkinson’s disease model composed of 3D bioprinted dopaminergic neurons within a biomimetic peptide scaffold. Biofabrication, 14: 044103. https://doi.org/10.1088/1758-5090/ac7eec
- Domingos M, Intranuovo F, Russo T, et al., 2013, The first systematic analysis of 3D rapid prototyped poly(ε- caprolactone) scaffolds manufactured through BioCell printing: The effect of pore size and geometry on compressive mechanical behaviour and in vitro hMSC viability. Biofabrication, 5: 045004. https://doi.org/10.1088/1758-5082/5/4/045004
- Lee JW, Ahn G, Kim JY, et al., 2010, Evaluating cell proliferation based on internal pore size and 3D scaffold architecture fabricated using solid freeform fabrication technology. J Mater Sci Mater Med, 21: 3195–3205. https://doi.org/10.1007/s10856-010-4173-7
- Sobral JM, Caridade SG, Sousa RA, et al., 2011, Three-dimensional plotted scaffolds with controlled pore size gradients: Effect of scaffold geometry on mechanical performance and cell seeding efficiency. Acta Biomater, 7: 1009–1018. https://doi.org/10.1016/j.actbio.2010.11.003
- Schuller T, Fanzio P, Galindo-Rosales FJ, 2022, Analysis of the importance of shear-induced elastic stresses in material extrusion. Addit Manuf, 57: 102952. https://doi.org/10.1016/j.addma.2022.102952
- Skylar-Scott MA, Mueller J, Visser CW, et al., 2019, Voxelated soft matter via multimaterial multinozzle 3D printing. Nature, 575: 330–335. https://doi.org/10.1038/s41586-019-1736-8
- Albalawi HI, Khan ZN, Rawas RH, et al., 2023, 3D-printed disposable nozzles for cost-efficient extrusion-based 3D bioprinting. Mater Sci Addit Manuf, 2: 52. https://doi.org/10.36922/msam.52
- Susapto HH, Alhattab D, Abdelrahman S, et al., 2021, Ultrashort peptide bioinks support automated printing of large-scale constructs assuring long-term survival of printed tissue constructs. Nano Lett, 21: 2719–2729. https://doi.org/10.1021/acs.nanolett.0c04426
- Alhattab D, Jamali F, Ali D, et al., 2019, An insight into the whole transcriptome profile of four tissue-specific human mesenchymal stem cells. Regen Med, 14: 841–865. https://doi.org/10.2217/rme-2018-0137
- Zhang Y, Liu Y, Xu Z, et al., 2020, Nucleation-controlled growth of superior lead-free perovskite Cs3Bi2I9 single-crystals for high-performance X-ray detection. Nat Commun, 11: 2304. https://doi.org/10.1038/s41467-020-16034-w
- Gungor-Ozkerim PS, Inci I, Zhang YS, et al., 2018, Bioinks for 3D bioprinting: An overview. Biomater Sci, 6: 915–946. https://doi.org/10.1039/c7bm00765e
- Silva C, Cortés-Rodriguez CJ, Hazur J, et al., 2020, Rational design of a triple-layered coaxial extruder system: In silico and in vitro evaluations directed toward optimizing cell viability. Int J Bioprint, 6: 282. https://doi.org/10.18063/ijb.v6i4.282
- Shi J, Wu B, Li S, et al., 2018, Shear stress analysis and its effects on cell viability and cell proliferation in drop-on-demand bioprinting. Biomed Phys Eng Express, 4: 045028. https://doi.org/10.1088/2057-1976/aac946
- Alhattab DM, Khan Z, Alshehri S, et al., 2023, 3D bioprinting of ultrashort self-assembling peptides to engineer scaffolds with different matrix stiffness for chondrogenesis. Int J Bioprint, 9: 719. https://doi.org/10.18063/ijb.719
- Rauf S, Susapto HH, Kahin K, et al., 2021, Self-assembling tetrameric peptides allow in situ 3D bioprinting under physiological conditions. J Mater Chem B, 9: 1069–1081. https://doi.org/10.1039/d0tb02424d
- Daly AC, Prendergast ME, Hughes AJ, et al., 2021, Bioprinting for the biologist. Cell, 184: 18–32. https://doi.org/10.1016/j.cell.2020.12.002
- Sohn S, Van Buskirk M, Buckenmeyer MJ, et al., 2020, Whole organ engineering: Approaches, challenges, and future directions. Appl Sci, 10: 4277. https://doi.org/10.3390/app10124277