Direct ink writing of biomimetic hydroxyapatite scaffolds with tailored concave porosity
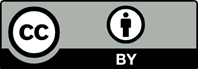
Direct ink writing (DIW) is a promising technology for the fabrication of personalized bone grafts, as it enables the customization of their geometrical conformation with high reproducibility and is compatible with the use of self-setting calcium-deficient hydroxyapatite inks. However, the scaffolds obtained by DIW consist mostly of convex filaments, which is a limitation since concave surfaces are known to promote bone regeneration in vivo. In this work, we explore the use of triply periodic minimal surface (TPMS) designs in DIW of calcium phosphate self-hardening inks as a strategy to obtain scaffolds with controlled concave macropores. The limitations of the printing parameters with high ceramic-loaded inks using DIW resulted in only 20% nominal porosity for gyroid-, diamond-, and Schwarz-based structures. The inherent layered pores from TPMS geometries enabled concavities typically unattainable via DIW, bearing substantial implications for subsequent osteoinductive capabilities. Although the mechanical properties were lower in the TPMS-based scaffolds than in the orthogonal patterned ones, the blood permeability of TPMS-based structures was higher. The concave pore architecture enhanced the osteogenic potential of the biomimetic ceramic, increasing SaOs-2 cell adhesion, proliferation, differentiation, and mineralization.

- Kalita SJ, Bose S, Hosick HL, Bandyopadhyay A. Development of controlled porosity polymer-ceramic composite scaffolds via fused deposition modeling. Mater Sci Eng C. 2003;23:611-620. doi: 10.1016/S0928-4931(03)00052-3
- Bohner M, Galea L, Doebelin N. Calcium phosphate bone graft substitutes: failures and hopes. J Eur Ceram Soc. 2012;32:2663-2671. doi: 10.1016/j.jeurceramsoc.2012.02.028
- Perez RA, Mestres G. Role of pore size and morphology in musculo-skeletal tissue regeneration. Mater Sci Eng C. 2016;61:922-939. doi: 10.1016/J.MSEC.2015.12.087
- Habraken W, Habibovic P, Epple M, Bohner M. Calcium phosphates in biomedical applications: materials for the future? Mater Today. 2016;19:69-87. doi: 10.1016/J.MATTOD.2015.10.008
- Bose S, Vahabzadeh S, Bandyopadhyay A. Bone tissue engineering using 3D printing. Mater Today. 2013;16:496-504. doi: 10.1016/J.MATTOD.2013.11.017
- Thavornyutikarn B, Chantarapanich N, Sitthiseripratip K, Thouas GA, Chen Q. Bone tissue engineering scaffolding: computer-aided scaffolding techniques. Prog Biomater. 2014;3:61-102. doi: 10.1007/s40204-014-0026-7
- Bohner M, Baroud G, Bernstein A, et al. Characterization and distribution of mechanically competent mineralized tissue in micropores of β-tricalcium phosphate bone substitutes. Mater Today. 2017;20:106-115. doi: 10.1016/j.mattod.2017.02.002
- Graziano A, D’Aquino R, Angelis MGC-D, et al. Scaffold’s surface geometry significantly affects human stem cell bone tissue engineering. J Cell Physiol. 2008;214:166-172. doi: 10.1002/jcp.21175
- Barba A, Diez-Escudero A, Maazouz Y, et al. Osteoinduction by foamed and 3D-printed calcium phosphate scaffolds: effect of nanostructure and pore architecture. ACS Appl Mater Interfaces. 2017;9:41722-41736. doi: 10.1021/acsami.7b14175
- Barba A, Diez-Escudero Y, Espanol M, et al. Impact of biomimicry in the design of osteoinductive bone substitutes: nanoscale matters. ACS Appl Mater Interfaces. 2019; 11:8818–8830. doi: 10.1021/acsami.8b20749
- Ginebra MP, Espanol M, Montufar EB, Perez RA, Mestres G. New processing approaches in calcium phosphate cements and their applications in regenerative medicine. Acta Biomater. 2010;6:2863-2873. doi: 10.1016/J.ACTBIO.2010.01.036
- Jinnai H, Watashiba H, Kajihara T, Nishikawa Y, Takahashi M, Ito M. Surface curvatures of trabecular bone microarchitecture. Bone. 2002;30:191-194. doi: 10.1016/S8756-3282(01)00672-X
- Shen M, Li Y, Lu F, et al. Bioceramic scaffolds with triply periodic minimal surface architectures guide early-stage bone regeneration. Bioact Mater. 2023;25:374-386. doi: 10.1016/j.bioactmat.2023.02.012
- Blanquer SBG, Werner M, Hannula M, et al. Surface curvature in triply-periodic minimal surface architectures as a distinct design parameter in preparing advanced tissue engineering scaffolds. Biofabrication. 2017;9:025001. doi: 10.1088/1758-5090/aa6553
- Restrepo S, Ocampo S, Ramirez JA, Paucar C, Garcia C. Mechanical properties of ceramic structures based on Triply Periodic Minimal Surface (TPMS) processed by 3D printing. J Phys Conf Ser. 2017;935:0-6.v doi: 10.1088/1742-6596/935/1/012036
- Montazerian H, Mohamed MGA, Montazeri MM, et al. Permeability and mechanical properties of gradient porous PDMS scaffolds fabricated by 3D-printed sacrificial templates designed with minimal surfaces. Acta Biomater. 2019;96:149-160. doi: 10.1016/j.actbio.2019.06.040
- Montazerian H, Zhianmanesh M, Davoodi E, Milani AS, Hoorfar M. Longitudinal and radial permeability analysis of additively manufactured porous scaffolds: effect of pore shape and porosity. Mater Des. 2017;122:146-156. doi: 10.1016/j.matdes.2017.03.006
- Ali D, Ozalp M, Blanquer SBG, Onel S. Permeability and fluid flow-induced wall shear stress in bone scaffolds with TPMS and lattice architectures: a CFD analysis. Eur J Mech B/Fluids. 2020;79:376-385. doi: 10.1016/j.euromechflu.2019.09.015
- Diez-Escudero A, Harlin H, Isaksson P, Persson C. Porous polylactic acid scaffolds for bone regeneration: a study of additively manufactured triply periodic minimal surfaces and their osteogenic potential. J Tissue Eng. 2020;11:2041731420956541. doi: 10.1177/2041731420956541
- Castro APG, Pires T, Santos JE, Gouveia BP, Fernandes PR. Permeability versus design in TPMS scaffolds. Materials (Basel). 2019;12:1313. doi: 10.3390/ma12081313
- Castro APG, Ruben RB, Gonçalves SB, Pinheiro J, Guedes JM, Fernandes PR. Numerical and experimental evaluation of TPMS Gyroid scaffolds for bone tissue engineering. Comput Methods Biomech Biomed Engin. 2019;22: 567-573. doi: 10.1080/10255842.2019.1569638
- Maskery I, Sturm L, Aremu AO, et al. Insights into the mechanical properties of several triply periodic minimal surface lattice structures made by polymer additive manufacturing. Polymer (Guildf). 2018; 152:62–71. doi: 10.1016/j.polymer.2017.11.049
- Yan C, Hao L, Hussein A, Young P. Ti-6Al-4V triply periodic minimal surface structures for bone implants fabricated via selective laser melting. J Mech Behav Biomed Mater. 2015;51:61-73. doi: 10.1016/j.jmbbm.2015.06.024
- Bobbert FSL, Lietaert K, Eftekhari AA, et al. Additively manufactured metallic porous biomaterials based on minimal surfaces: a unique combination of topological, mechanical, and mass transport properties. Acta Biomater. 2017;53:572-584. doi: 10.1016/j.actbio.2017.02.024
- Kleger N, Fehlmann S, Lee SS, et al. Light-based printing of leachable salt molds for facile shaping of complex structures. Adv Mater. 2022;34(32):e2203878. doi: 10.1002/adma.202203878
- Tikhonov A, Evdokimov P, Klimashina E, et al. Putlayev, stereolithographic fabrication of three-dimensional permeable scaffolds from CaP/PEGDA hydrogel biocomposites for use as bone grafts. J Mech Behav Biomed Mater. 2020;110:103922. doi: 10.1016/j.jmbbm.2020.103922
- Paré A, Charbonnier B, Tournier P, et al. Tailored three-dimensionally printed triply periodic calcium phosphate implants: a preclinical study for craniofacial bone repair. ACS Biomater Sci Eng. 2020;6:553-563. doi: 10.1021/acsbiomaterials.9b01241
- Zhang Q, Ma L, Ji X, et al. High-strength hydroxyapatite scaffolds with minimal surface macrostructures for load-bearing bone regeneration. Adv Funct Mater. 2022;32:1-12. doi: 10.1002/adfm.202204182
- Bouakaz I, Drouet C, Grossin D, Cobraiville E, Nolesn G. Hydroxyapatite 3D-printed scaffolds with Gyroid-TPMS porous structure: fabrication and in vivo pilot study in sheep. Acta Biomater. 2023;170:580-595. doi: 10.1016/j.actbio.2023.08.041
- Deng ZL, Pan MZ, Bin Hua S, Wu JM, Zhang XY, Shi YS. Mechanical and degradation properties of triply periodic minimal surface (TPMS) hydroxyapatite & akermanite scaffolds with functional gradient structure. Ceram Int. 2023;49:20808–20816. doi: 10.1016/j.ceramint.2023.03.213
- Roohani I, Entezari A, Zreiqat H. Liquid crystal display technique (LCD) for high resolution 3D printing of triply periodic minimal surface lattices bioceramics. Addit Manuf. 2023;74:103720. doi: 10.1016/j.addma.2023.103720
- Bin Hua S, Yuan X, Wu JM, et al. Digital light processing porous TPMS structural HA & akermanite bioceramics with optimized performance for cancellous bone repair. Ceram Int. 2022;48:3020-3029. doi: 10.1016/j.ceramint.2021.10.003
- Oftadeh R, Perez-Viloria M, Villa-Camacho JC, Vaziri A, Nazarian A. Biomechanics and mechanobiology of trabecular bone: a review. J Biomech Eng. 2015;137:1–15. doi: 10.1115/1.4029176
- del-Mazo-Barbara L, Ginebra MP. Rheological characterisation of ceramic inks for 3D direct ink writing: a review. J Eur Ceram Soc. 2021;41:18-33. doi: 10.1016/J.JEURCERAMSOC.2021.08.031
- Raymond Y, Johansson L, Thorel E, Ginebra MP. Translation of three-dimensional printing of ceramics in bone tissue engineering and drug delivery. MRS Bull. 2022;47:59-69. doi: 10.1557/s43577-021-00259-1
- Maazouz Y, Montufar EB, Guillem-Marti J, et al. Robocasting of biomimetic hydroxyapatite scaffolds using self-setting inks. J Mater Chem B. 2014;2:5378-5386. doi: 10.1039/C4TB00438H
- Raymond S, Maazouz Y, Montufar EB, et al. Accelerated hardening of nanotextured 3D-plotted self-setting calcium phosphate inks. Acta Biomater. 2018;75:451-462. doi: 10.1016/j.actbio.2018.05.042
- Konka J, Buxadera-Palomero J, Espanol M, Ginebra MP. 3D printing of hierarchical porous biomimetic hydroxyapatite scaffolds: adding concavities to the convex filaments. Acta Biomater. 2021;134:744-759. doi: 10.1016/j.actbio.2021.07.071
- Raymond Y, Bonany M, Lehmann C, et al. Hydrothermal processing of 3D-printed calcium phosphate scaffolds enhances bone formation in vivo: a comparison with biomimetic treatment. Acta Biomater. 20221; 135:671-688. doi: 10.1016/j.actbio.2021.09.001
- Raymond Y, Thorel E, Liversain M, Riveiro A, Pou J, Ginebra MP. 3D printing non-cylindrical strands: morphological and structural implications. Addit Manuf. 2021;46: 102129. doi: 10.1016/j.addma.2021.102129
- Moreno Madrid AP, Vrech SM, Sanchez MA, Rodriguez AP. Advances in additive manufacturing for bone tissue engineering scaffolds. Mater Sci Eng C. 2019;100: 631-644. doi: 10.1016/j.msec.2019.03.037
- Roopavath UK, Malferrari S, Van Haver A, Verstreken SM, Rath SN, Kalaskar DM. Optimization of extrusion based ceramic 3D printing process for complex bony designs. Mater Des. 2019;162:263-270. doi: 10.1016/j.matdes.2018.11.054
- Shao H, He J, Lin T, Zhang Z, Zhang Y, Liu S. 3D gel-printing of hydroxyapatite scaffold for bone tissue engineering. Ceram Int. 2019;45:1163-1170. doi: 10.1016/j.ceramint.2018.09.300
- Wang Y, Chen S, Liang H, Liu Y, Bai J, Wang M. Digital light processing (DLP) of nano biphasic calcium phosphate bioceramic for making bone tissue engineering scaffolds. Ceram Int. 2022;48:27681-27692. doi: 10.1016/j.ceramint.2022.06.067
- Navarrete-Segado P, Tourbin M, Frances C, Grossin D. Masked stereolithography of hydroxyapatite bioceramic scaffolds: from powder tailoring to evaluation of 3D printed parts properties. Open Ceram. 2022;9:100235. doi: 10.1016/j.oceram.2022.100235
- Ginebra M-P, Espanol M, Maazouz Y, Bergez V, Pastorino D. Bioceramics and bone healing. EFORT Open Rev. 2018;3:173-183. doi: 10.1302/2058-5241.3.170056
- Vidal L, Kampleitner C, Krissian S, et al. Regeneration of segmental defects in metatarsus of sheep with vascularized and customized 3D-printed calcium phosphate scaffolds. Sci Rep. 2020;10:7068. doi: 10.1038/s41598-020-63742-w
- Feng C, Zhang W, Deng C, et al. 3D printing of lotus root-like biomimetic materials for cell delivery and tissue regeneration. Adv Sci (Weinh). 2017;4(12):1700401. doi: 10.1002/advs.201700401
- Raymond Y, Lehmann C, Thorel E, et al. 3D printing with star-shaped strands: a new approach to enhance in vivo bone regeneration. Biomater Adv. 2022;137:12807. doi: 10.1016/j.bioadv.2022.212807
- Moon YW, Choi IJ, Koh YH, Kim C. Porous alumina ceramic scaffolds with biomimetic macro/micro-porous structure using three-dimensional (3-D) ceramic/camphene-based extrusion. Ceram Int. 2015;41:12371-12377. doi: 10.1016/j.ceramint.2015.06.069
- Minas C, Carnelli D, Tervoort E, Studart AR. 3D printing of emulsions and foams into hierarchical porous ceramics. Adv Mater. 2016;28:9993-9999. doi: 10.1002/adma.201603390
- Ginebra MP, Fernández E, De Maeyer, et al. Setting reaction and hardening of an apatitic calcium phosphate cement. J Dent Res. 1997;76:905-912. doi: 10.1177/00220345970760041201
- Brookshier KA, Tarbell JM. Evaluation of a transparent blood analog fluid: aqueous Xanthan gum/glycerin. Biorheology. 1993;30:107-116. doi: 10.3233/BIR-1993-30202
- Chan SSL, Sesso ML, Franks GV. Direct ink writing of hierarchical porous alumina-stabilized emulsions: rheology and printability. J Am Ceram Soc. 2020;103:5554-5566. doi: 10.1111/jace.17305
- Seoane-Viaño I, Januskaite P, Alvarez-Lorenzo C, Basit AW, Goyanes A. Semi-solid extrusion 3D printing in drug delivery and biomedicine: personalised solutions for healthcare challenges. J Control Release. 2021;332:367-389. doi: 10.1016/j.jconrel.2021.02.027
- Franco ESJ, Hunger P, Launey ME, Tomsia AP. Direct-write assembly of calcium phosphate scaffolds using a water-based hydrogel. Acta Biomater. 2010;6:218-228. doi: 10.1016/j.actbio.2009.06.031.Direct-Write
- Mys K, Varga P, Stockmans F, et al. Quantification of 3D microstructural parameters of trabecular bone is affected by the analysis software. Bone. 2021;142:115653. doi: 10.1016/j.bone.2020.115653
- Maazouz Y, Montufar EB, Malbert J, Espanol M, Ginebra MP. Self-hardening and thermoresponsive alpha tricalcium phosphate/pluronic pastes. Acta Biomater. 2017;49:563-574. doi: 10.1016/j.actbio.2016.11.043
- Barba A, Maazouz Y, Diez-Escudero A, et al. Osteogenesis by foamed and 3D-printed nanostructured calcium phosphate scaffolds: effect of pore architecture. Acta Biomater. 2018;79:135-147. doi: 10.1016/j.actbio.2018.09.003
- Anastasiou AD, Spyrogianni AS, Koskinas KC, Giannoglou GD, Paras SV. Experimental investigation of the flow of a blood analogue fluid in a replica of a bifurcated small artery. Med Eng Phys. 2012;34(2):211-218. doi: 10.1016/j.medengphy.2011.07.012
- Webb L. Mimicking blood rheology for more accurate modeling in benchtop research. Pegasus Rev UCF Undergrad Res J. 2020;12:28-34. https://stars.library.ucf.edu/urj/vol12/iss1/6
- Alves MM, Rocha C, Gonçalves MP. Study of the rheological behaviour of human blood using a controlled stress rheometer. Clin Hemorheol Microcirc. 2013;53:369-386. doi: 10.3233/ch-121645
- Entezari A, Liu NC, Roohani I, et al. On design for additive manufacturing (DAM) parameter and its effects on biomechanical properties of 3D printed ceramic scaffolds. Mater Today Commun. 2020;23:101065. doi: 10.1016/j.mtcomm.2020.101065
- Miranda P, Pajares A, Saiz E, Tomsia AP, Guiberteau F. Mechanical properties of calcium phosphate scaffolds fabricated by robocasting. J Biomed Mater Res A. 2008;85:218-227. doi: 10.1002/jbm.a.31587
- Charbonnier B, Manassero M, Bourguignon M, et al. Custom-made macroporous bioceramic implants based on triply-periodic minimal surfaces for bone defects in load-bearing sites. Acta Biomater. 2020;109:254-266. doi: 10.1016/j.actbio.2020.03.016
- Panzavolta S, Torricelli P, Sturba L, Bracci B, Giardino R, Bigi A. Setting properties and in vitro bioactivity of strontium-enriched gelatin-calcium phosphate bone cements. J Biomed Mater Res A. 2008;84:965-972. doi: 10.1002/jbm.a.31412
- Sadowska JM, Guillem-Marti J, Ginebra MP. The influence of physicochemical properties of biomimetic hydroxyapatite on the in vitro behavior of endothelial progenitor cells and their interaction with mesenchymal stem cells. Adv Healthc Mater. 2019;8(2):e1801138. doi: 10.1002/adhm.201801138
- Farley JR, Hall SL, Tanner MA, Wergedal JE. Specific activity of skeletal alkaline phosphatase in human osteoblast-line cells regulated by phosphate, phosphate esters, and phosphate analogs and release of alkaline phosphatase activity inversely regulated by calcium. J Bone Miner Res. 1994;9(4):497-508. doi: 10.1002/jbmr.5650090409
- Gao L, McBeath R, Chen CS. Stem cell shape regulates a chondrogenic versus myogenic fate through Rac1 and N-cadherin. Stem Cells. 2010;28(3):564-572. doi: 10.1002/stem.308
- Sugawara Y, Suzuki K, Koshikawa M, Ando M, Iida J. Necessity of enzymatic activity of alkaline phosphatase for mineralization of osteoblastic cells. Jpn J Pharmacol. 2002;88(3):262-269. doi: 10.1254/jjp.88.262
- Bianchi M, Urquia Edreira ER, Wolke JG, et al. Substrate geometry directs the in vitro mineralization of calcium phosphate ceramics. Acta Biomater. 2014;10(2):661-669. doi: 10.1016/j.actbio.2013.10.026
- Urquia Edreira ER, Wolke JG, Jansen JA, van den Beucken JJ. Influence of ceramic disk material, surface hemispheres, and SBF volume on in vitro mineralization. J Biomed Mater Res A. 2015;103(8): 2740-2746. doi: 10.1002/jbm.a.35406