Considering cell viability in 3D printing of structured inks: A comparative and equivalent analysis of fluid forces
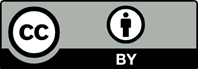
In conventional extrusion-based three-dimensional (3D) printing (E3DP), smaller needles reduce cell viability due to increased fluid forces like pressure and shear stress. A novel E3DP approach has emerged, involving 3D printing with structured inks. Fluid forces in both conventional and structured ink-based methods were evaluated through computational fluid dynamics (CFD) simulations. By employing 18G needles, we showcased the advantages of structured inks, including 2-symmetric, 4-symmetric, vascular-like, and hepatic lobule analogue-like inks, which demonstrated consistently lower pressures and shear stress compared with conventional inks. Specifically, vascular-like inks with a 2:1:1 extruded fiber layer distance showed significantly lower shear stress (average 6.595e+0 Pa, maximum 2.069e+2 Pa) than conventional methods. Equivalent analyses explored commonly used symmetric and core–shell inks, examining fluid forces on cells. Particularly, in core–shell inks with a 2.8 mm core layer radius, cells in the flow domain of the shell layer experienced an equivalent viscosity of 3.70 Pa·s, while in the core layer, it was 1.72 Pa·s. The analyses revealed a positive correlation between equivalent homogeneous ink viscosity and shear stress. The proposed workflow, emphasizing cell viability, offers an efficient approach for structured ink design. Also, experiments that used vascular-like ink-based printing as an example indicated significantly higher cell viability when compared with conventional printing. This research provides valuable insights for enhancing cell viability in 3D printing and advancing printing material design.
- Pacheco DP, Vargas NS, Visentin S, Petrini P. From tissue engineering to engineering tissues: the role and application of in vitro models. Biomater Sci. 2021;9(1):70-83. doi: 10.1039/D0BM01097A
- Huskin G, Chen J, Davis T, Jun H-W. Tissue-engineered 3D in vitro disease models for high-throughput drug screening. Tissue Eng Regen Med. 2023;20(4):523-528. doi: 10.1007/s13770-023-00522-3
- Gaharwar AK, Singh I, Khademhosseini A. Engineered biomaterials for in situ tissue regeneration. Nat Rev Mater. 2020;5(9):686-705. doi: 10.1038/s41578-020-0209-x
- Huang G, Li F, Zhao X, et al. Functional and biomimetic materials for engineering of the three-dimensional cell microenvironment. Chem Rev. 2017;117(20):12764-12850. doi: 10.1021/acs.chemrev.7b00094
- Ullah S, Chen X. Fabrication, applications and challenges of natural biomaterials in tissue engineering. Appl Mater Today. 2020;20:100656. doi: 10.1016/j.apmt.2020.100656
- Wang P, Sun Y, Shi X, Shen H, Ning H, Liu H. Bioscaffolds embedded with regulatory modules for cell growth and tissue formation: a review. Bioact Mater. 2021;6(5): 1283-1307. doi: 10.1016/j.bioactmat.2020.10.014
- Lepowsky E, Muradoglu M, Tasoglu S. Towards preserving post-printing cell viability and improving the resolution: past, present, and future of 3D bioprinting theory. Bioprinting. 2018;11:e00034. doi: 10.1016/j.bprint.2018.e00034
- Knight E, Przyborski S. Advances in 3D cell culture technologies enabling tissue‐like structures to be created in vitro. J Anat. 2015;227(6):746-756. doi: 10.1111/joa.12257
- Brett E, Chung N, Leavitt WT, Momeni A, Longaker MT, Wan DC. A review of cell-based strategies for soft tissue reconstruction. Tissue Eng Part B Rev. 2017;23(4):336-346. doi: 10.1089/ten.teb.2016.0455
- Wang P, Sun Y, Shi X, Shen H, Ning H, Liu H. 3D printing of tissue engineering scaffolds: a focus on vascular regeneration. Bio-Des Manuf. 2021;4(3):344-378. doi: 10.1007/s42242-020-00109-0
- Fang Y, Ouyang L, Zhang T, Wang C, Lu B, Sun W. Optimizing bifurcated channels within an anisotropic scaffold for engineering vascularized oriented tissues. Adv Healthc Mater. 2020;9(24):2000782. doi: 10.1002/adhm.202000782
- Wang T, Li W, Zhang Y, et al. Bioprinted constructs that simulate nerve–bone crosstalk to improve microenvironment for bone repair. Bioact Mater. 2023;27:377-393. doi: 10.1016/j.bioactmat.2023.02.013
- He Y, Xie M, Gao Q, Fu J. Why choose 3D bioprinting? Part I: a brief introduction of 3D bioprinting for the beginners. Bio-Des Manuf. 2019;2(4):221-224. doi: 10.1007/s42242-019-00053-8
- Zhang L, Wang B, Song B, et al. 3D printed biomimetic metamaterials with graded porosity and tapering topology for improved cell seeding and bone regeneration. Bioact Mater. 2023;25:677-688. doi: 10.1016/j.bioactmat.2022.07.009
- Zieliński PS, Gudeti PKR, Rikmanspoel T, Włodarczyk- Biegun MK. 3D printing of bio-instructive materials: toward directing the cell. Bioact Mater. 2023;19:292-327. doi: 10.1016/j.bioactmat.2022.04.008
- Thakare K, Jerpseth L, Qin H, Pei Z. Bioprinting using algae: effects of extrusion pressure and needle diameter on cell quantity in printed samples. J Manuf Sci Eng. 2021;143(1):014501. doi: 10.1115/1.4048853
- Choi DJ, Kho Y, Park SJ, Kim Y-J, Chung S, Kim C-H. Effect of cross-linking on the dimensional stability and biocompatibility of a tailored 3D-bioprinted gelatin scaffold. Int J Biol Macromol. 2019;135:659-667. doi: 10.1016/j.ijbiomac.2019.05.207
- Huh J, Moon Y-W, Park J, Atala A, Yoo JJ, Lee SJ. Combinations of photoinitiator and UV absorber for cell-based digital light processing (DLP) bioprinting. Biofabrication. 2021;13(3):034103. doi: 10.1088/1758-5090/abfd7a
- Saroia J, Yanen W, Wei Q, Zhang K, Lu T, Zhang B. A review on biocompatibility nature of hydrogels with 3D printing techniques, tissue engineering application and its future prospective. Bio-Des Manuf. 2018;1(4):265-279. doi: 10.1007/s42242-018-0029-7
- De France KJ, Xu F, Hoare T. Structured macroporous hydrogels: progress, challenges, and opportunities. Adv Healthc Mater. 2018;7(1):1700927. doi: 10.1002/adhm.201700927
- Gun’ko VM, Savina IN, Mikhalovsky SV. Properties of water bound in hydrogels. Gels. 2017;3(4):37. doi: 10.3390/gels3040037
- Santoni S, Gugliandolo SG, Sponchioni M, Moscatelli D, Colosimo BM. 3D bioprinting: current status and trends—a guide to the literature and industrial practice. Bio-Des Manuf. 2022;5(1):14-42. doi: 10.1007/s42242-021-00165-0
- Shao L, Gao Q, Xie C, et al. Sacrificial microgel-laden bioink-enabled 3D bioprinting of mesoscale pore networks. Bio-Des Manuf. 2020;3(1):30-39. doi: 10.1007/s42242-020-00062-y
- Nikolopoulos VK, Augustine R, Camci-Unal G. Harnessing the potential of oxygen-generating materials and their utilization in organ-specific delivery of oxygen. Biomater Sci. 2023;11(5):1567-1588. doi: 10.1039/D2BM01329K
- Ouyang L, Wojciechowski JP, Tang J, Guo Y, Stevens MM. Tunable microgel‐templated porogel (MTP) bioink for 3D bioprinting applications. Adv Healthc Mater. 2022;11(8):2200027. doi: 10.1002/adhm.202200027
- Wang X, Yang C, Yu Y, Zhao Y. In situ 3D bioprinting living photosynthetic scaffolds for autotrophic wound healing. Research. 2022;2022:1-11. doi: 10.34133/2022/9794745
- Blaeser A, Duarte Campos DF, Puster U, Richtering W, Stevens MM, Fischer H. Controlling shear stress in 3D bioprinting is a key factor to balance printing resolution and stem cell integrity. Adv Healthc Mater. 2016;5(3):326-333. doi: 10.1002/adhm.201500677
- Wang P, Sun Y, Ma Z, Diao L, Liu H, Shastri VP. Novel stirring-rod-inspired mixer-integrated printhead for fabricating gradient tissue structures. Mater Design. 2023;229:111866. doi: 10.1016/j.matdes.2023.111866
- Ouyang L. Pushing the rheological and mechanical boundaries of extrusion-based 3D bioprinting. Trends Biotechnol. 2022;40(7):891-902. doi: 10.1016/j.tibtech.2022.01.001
- Wang P, Sun Y, Li D, et al. Extrusion-based 3D co-printing: printing material design and novel workflow for fabricating patterned heterogeneous tissue structures. Mater Design. 2023;227:111737. doi: 10.1016/j.matdes.2023.111737
- Kang D, Ahn G, Kim D, et al. Pre-set extrusion bioprinting for multiscale heterogeneous tissue structure fabrication. Biofabrication. 2018;10(3):035008. doi: 10.1088/1758-5090/aac70b
- Manoj Prabhakar M, Saravanan AK, Haiter Lenin A, Jerin leno I, Mayandi K, Sethu Ramalingam P. A short review on 3D printing methods, process parameters and materials. Mater Today Proc. 2021;45:6108-6114. doi: 10.1016/j.matpr.2020.10.225
- Ates G, Bartolo P. Computational fluid dynamics for the optimization of internal bioprinting parameters and mixing conditions. Int J Bioprinting. 2023;9(6):0219. doi: 10.36922/ijb.0219
- Samandari M, Alipanah F, Majidzadeh-A K, Alvarez MM, Santiago GT, Tamayol A. Controlling cellular organization in bioprinting through designed 3D microcompartmentalization. Appl Phys Rev. 2021;8(2):021404. doi: 10.1063/5.0040732
- Gao Q, Liu Z, Lin Z, et al. 3D bioprinting of vessel-like structures with multilevel fluidic channels. ACS Biomater Sci Eng. 2017;3(3):399-408. doi: 10.1021/acsbiomaterials.6b00643
- Kang D, Hong G, An S, et al. Bioprinting of multiscaled hepatic lobules within a highly vascularized construct. Small. 2020;16(13):1905505. doi: 10.1002/smll.201905505
- Ershkov SV, Prosviryakov EY, Burmasheva NV, Christianto V. Towards understanding the algorithms for solving the Navier–Stokes equations. Fluid Dyn Res. 2021;53(4):044501. doi: 10.1088/1873-7005/ac10f0
- Zhang X, Kim GJ, Kang MG, et al. Marine biomaterial-based bioinks for generating 3D printed tissue constructs. Mar Drugs. 2018;16(12):484. doi: 10.3390/md16120484
- Chand R, Muhire BS, Vijayavenkataraman S. Computational fluid dynamics assessment of the effect of bioprinting parameters in extrusion bioprinting. Int J Bioprinting. 2022;8(2):545. doi: 10.18063/ijb.v8i2.545
- Caccavo D, Cascone S, Lamberti G, Barba AA. Hydrogels: experimental characterization and mathematical modelling of their mechanical and diffusive behaviour. Chem Soc Rev. 2018;47(7):2357-2373. doi: 10.1039/C7CS00638A
- Gutierrez RA, Crumpler ET. Potential effect of geometry on wall shear stress distribution across scaffold surfaces. Ann Biomed Eng. 2008;36(1):77-85. doi: 10.1007/s10439-007-9396-5
- Rademakers T, Horvath JM, van Blitterswijk CA, LaPointe VLS. Oxygen and nutrient delivery in tissue engineering: approaches to graft vascularization. J Tissue Eng Regen Med. 2019;13(10):1815-1829. doi: 10.1002/term.2932
- Liang Q, Gao F, Zeng Z, et al. Coaxial scale‐up printing of diameter‐tunable biohybrid hydrogel microtubes with high strength, perfusability, and endothelialization. Adv Funct Mater. 2020;30(43):2001485. doi: 10.1002/adfm.202001485
- Mirdamadi ES, Kalhori D, Zakeri N, Azarpira N, Solati- Hashjin M. Liver tissue engineering as an emerging alternative for liver disease treatment. Tissue Eng Part B Rev. 2020;26(2):145-163. doi: 10.1089/ten.teb.2019.0233
- Lv W, Zhou H, Aazmi A, et al. Constructing biomimetic liver models through biomaterials and vasculature engineering. Regen Biomater. 2022;9:rbac079. doi: 10.1093/rb/rbac079
- Son J, Hong SJ, Lim JW, Jeong W, Jeong JH, Kang H-K. Engineering tissue‐specific, multiscale microvasculature with a capillary network for prevascularized tissue. Small Methods. 2021;5(10):2100632. doi: 10.1002/smtd.202100632
- Xu H-Q, Liu J-C, Zhang Z-Y, Xu C-X. A review on cell damage, viability, and functionality during 3D bioprinting. Military Med Res. 2022;9(1):70. doi: 10.1186/s40779-022-00429-5
- Heidari F, Saadatmand M, Simorgh S. Directly coaxial bioprinting of 3D vascularized tissue using novel bioink based on decellularized human amniotic membrane. Int J Biol Macromol. 2023;253:127041. doi: 10.1016/j.ijbiomac.2023.127041
- Ghahri T, Salehi Z, Aghajanpour S, et al. Development of osteon-like scaffold-cell construct by quadruple coaxial extrusion-based 3D bioprinting of nanocomposite hydrogel. Biomater Adv. 2023;145:213254. doi: 10.1016/j.bioadv.2022.213254
- Bliley JM, Shiwarski DJ, Feinberg AW. 3D-bioprinted human tissue and the path toward clinical translation. Sci Transl Med. 2022;14(666):eabo7047. doi: 10.1126/scitranslmed.abo7047
- Wang P, Wang S. Computer-aided CT image processing and modeling method for tibia microstructure. Bio-Des Manuf. 2020;3(1):71-82. doi: 10.1007/s42242-020-00063-x
- Ning L, Gil CJ, Hwang B, et al. Biomechanical factors in three-dimensional tissue bioprinting. Appl Phys Rev. 2020;7(4):041319. doi: 10.1063/5.0023206
- Eça L, Hoekstra M. A procedure for the estimation of the numerical uncertainty of CFD calculations based on grid refinement studies. J Comput Phys. 2014;262:104-130. doi: 10.1016/j.jcp.2014.01.006
- Ouyang L, Highley CB, Rodell CB, Sun W, Burdick JA. 3D printing of shear-thinning hyaluronic acid hydrogels with secondary cross-linking. ACS Biomater Sci Eng. 2016;2(10):1743-1751. doi: 10.1021/acsbiomaterials.6b00158