Sub-regional design of the bionic bone scaffolds using macrostructural topology
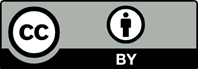
With the increasing demand for bone repair, the bionic bone scaffolds have become a research hotspot. A sub-regional design method of the bionic bone scaffolds, using macrostructural topology, is proposed in this paper, aiming to provide a functionally enhanced region division method for the gradient design. The macrostructural topology was carried out by the bi-directional evolutionary structural optimization (BESO), dividing the predefined design domain into sub-region A and sub-region B. Subsequently, a combined probability sphere model and a distance-to-scale coefficient mapping model are established to implement the graded porosification based on the Voronoi tessellation. This approach takes geometric and mechanical continuity into fully account and assures a reasonable distribution of characteristic parameters, yielding to improve the mechanical strength under specific stress conditions. Finally, the scaffolds were fabricated by the laser powder bed fusion (LPBF) process using the Ti-6Al-4V powder. The results of compression tests are satisfactory, showing that the as-built specimens implement sub-regional functionality. The apparent elastic modulus and the ultimate strength range, respectively, between 1.50 GPa and 7.12 GPa (for the first module) and between 38.55 MPa and 268.03 MPa (for the second module), which conform to the required level of natural bone, providing a possibility for clinical application.
- Pina S, Oliveira JM, Reis RL, 2015, Natural-based nanocomposites for bone tissue engineering and regenerative medicine: A review. Adv Mater, 27:1143–1169. https://doi.org/10.1002/adma.201403354
- Geetha M, Singh AK, Asokamani R, et al., 2009, Ti based biomaterials, the ultimate choice for orthopaedic implants—A review. Prog Mater Sci, 54:397–425. https://doi.org/10.1016/j.pmatsci.2008.06.004
- Choi K, Kuhn JL, Ciarelli MJ, et al., 1990, The elastic-moduli of human subchondral, trabecular, and cortical bone tissue and the size-dependency of cortical bone modulus. J Biomech, 23:1103–1113. https://doi.org/10.1016/0021-9290(90)90003-l
- Melancon D, Bagheri ZS, Johnston RB, et al., 2017, Mechanical characterization of structurally porous biomaterials built via additive manufacturing: experiments, predictive models, and design maps for load-bearing bone replacement implants. Acta Biomater, 63:350–368. https://doi.org/10.1016/j.actbio.2017.09.013
- Kantaros A, Chatzidai N, Karalekas D, 2016, 3D printing-assisted design of scaffold structures. Int J Adv Manuf Technol, 82:559–571. https://doi.org/10.1007/s00170-015-7386-6
- Tshephe TS, Akinwamide SO, Olevsky E, et al., 2022, Additive manufacturing of titanium-based alloys—A review of methods, properties, challenges, and prospects. Heliyon, 8:20. https://doi.org/10.1016/j.heliyon.2022.e09041
- Zhao D, Huang Y, Ao Y, et al., 2018, Effect of pore geometry on the fatigue properties and cell affinity of porous titanium scaffolds fabricated by selective laser melting. J Mech Behav Biomed Mater, 88:478–487. https://doi.org/10.1016/j.jmbbm.2018.08.048
- Yan X, Li Q, Yin S, et al., 2019, Mechanical and in vitro study of an isotropic Ti6Al4V lattice structure fabricated using selective laser melting, J Alloy Compd, 782:209–223. https://doi.org/10.1016/j.jallcom.2018.12.220
- Gomez S, Vlad MD, Lopez J, et al., 2016, Design and properties of 3D scaffolds for bone tissue engineering, Acta Biomater, 42:341–350. https://doi.org/10.1016/j.actbio.2016.06.032
- Rumpler M, Woesz A, Dunlop JWC, et al., 2008, The effect of geometry on three-dimensional tissue growth, J R Soc Interface, 5:1173–1180. https://doi.org/10.1098/rsif.2008.0064
- Biemond JE, Aquarius R, Verdonschot N, et al., 2011, Frictional and bone ingrowth properties of engineered surface topographies produced by electron beam technology. Arch Orthop Trauma Surg, 131:711–718. https://doi.org/10.1007/s00402-010-1218-9
- Wang G, Shen L, Zhao J, et al., 2018, Design and compressive behavior of controllable irregular porous scaffolds: Based on Voronoi-tessellation and for additive manufacturing. ACS Biomater Sci Eng, 4:719–727. https://doi.org/10.1021/acsbiomaterials.7b00916
- Du Y, Liang H, Xie D, et al., 2020, Design and statistical analysis of irregular porous scaffolds for orthopedic reconstruction based on Voronoi tessellation and fabricated via selective laser melting (SLM). Mater Chem Phys, 239:121968. https://doi.org/10.1016/j.matchemphys.2019.121968
- Van Bael S, Chai YC, Truscello S, et al., 2012, The effect of pore geometry on the in vitro biological behavior of human periosteum-derived cells seeded on selective laser-melted Ti6Al4V bone scaffolds. Acta Biomater, 8:2824–2834. https://doi.org/10.1016/j.actbio.2012.04.001
- Gongyi H, 2007, Characteristics of osteoporosis-related fracture and clinical study. Basic Clin Med, 27:1088–1092.
- Barbaros I, Yang YM, Safaei B, et al., 2022, State-of-the-art review of fabrication, application, and mechanical properties of functionally graded porous nanocomposite materials. Nanotechnol Rev, 11:321–371. https://doi.org/10.1515/ntrev-2022-0017
- Liu B, Chen H, Cao W, 2019, A novel method for tailoring elasticity distributions of functionally graded porous materials. Int J Mech Sci, 157–158:457–470. https://doi.org/10.1016/j.ijmecsci.2019.05.002
- George SM, Nayak C, Singh I, et al., 2022, Multifunctional hydroxyapatite composites for orthopedic applications: A review. ACS Biomater Sci Eng, 8:3162–3186. https://doi.org/10.1021/acsbiomaterials.2c00140
- Deering J, Dowling KI, DiCecco LA, et al., 2021, Selective Voronoi tessellation as a method to design anisotropic and biomimetic implants. J Mech Behav Biomed Mater, 116:104361. https://doi.org/10.1016/j.jmbbm.2021.104361
- Liu B, Cao W, Zhang L, et al., 2021, A design method of Voronoi porous structures with graded relative elasticity distribution for functionally gradient porous materials. Int J Mech Mater Des, 17:863–883. https://doi.org/10.1007/s10999-021-09558-6
- Davoodi E, Montazerian H, Mirhakimi AS, et al., 2022, Additively manufactured metallic biomaterials, Bioact Mater, 15:214–249. https://doi.org/10.1016/j.bioactmat.2021.12.027
- Zhou J, Xia L, Huang K, 2022, Design-oriented topology optimization of structures. J Ship Mech, 26:538–546.
- Xu FX, Zhang X, Zhang H, 2018, A review on functionally graded structures and materials for energy absorption. Eng Struct, 171:309–325.https://doi.org/10.1016/j.engstruct.2018.05.094
- Li H, Luo Z, Gao L, et al., 2018, Topology optimization for concurrent design of structures with multi-patch microstructures by level sets. Comput Meth Appl Mech Eng, 331:536–561. https://doi.org/10.1016/j.cma.2017.11.033
- Terriault P, Brailovski V, 2018, Modeling and simulation of large, conformal, porosity-graded and lightweight lattice structures made by additive manufacturing. Finite Elem Anal Des, 138:1–11. https://doi.org/10.1016/j.finel.2017.09.005
- Radman A, Huang X, Xie YM, 2012, Topology optimization of functionally graded cellular materials. J Mater Sci, 48:1503–1510. https://doi.org/10.1007/s10853-012-6905-1
- Alzahrani M, Choi SK, Rosen DW, 2015, Design of truss-like cellular structures using relative density mapping method. Mater Des, 85:349–360. https://doi.org/10.1016/j.matdes.2015.06.180
- Zhao F, 2018, Variable density cellular structure design method base on local relative density mapping. J Mech Eng, 54:121–8. https://doi.org/10.3901/jme.2018.19.121
- Wang C, Gu X, Zhu J, et al., 2020, Concurrent design of hierarchical structures with three-dimensional parameterized lattice microstructures for additive manufacturing. Struct Multidiscip O, 61:869–894. https://doi.org/10.1007/s00158-019-02408-2
- Xia L, Xia Q, Huang XD, et al., 2018, Bi-directional evolutionary structural optimization on advanced structures and materials: A comprehensive review. Arch Comput Method Eng, 25:437–478. https://doi.org/10.1007/s11831-016-9203-2
- Lee TU, Xie YM, 2022, Optimizing load locations and directions in structural design. Finite Elem Anal Des, 209:8. https://doi.org/10.1016/j.finel.2022.103811
32 Bi MH, Tran P, Xia LW, et al., 2022, Topology optimization for 3D concrete printing with various manufacturing constraints. Addit Manuf, 57:15. https://doi.org/10.1016/j.addma.2022.102982
- Han YS, Xu B, Duan ZY, et al., 2022, Stress-based topology optimization of continuum structures for the elastic contact problems with friction. Struct Multidiscip O, 65:22. https://doi.org/10.1007/s00158-022-03169-1
- Han YS, Xu B, Duan ZY, et al., 2022, Stress-based multi-material structural topology optimization considering graded interfaces. Comput Meth Appl Mech Eng, 391:31. https://doi.org/10.1016/j.cma.2022.114602
- Zhu L, Liang H, Lv F, et al., 2021, Design and compressive fatigue properties of irregular porous scaffolds for orthopedics fabricated using selective laser melting, ACS Biomater Sci Eng, 7:1663–1672. https://doi.org/10.1021/acsbiomaterials.0c01392
- Liang H, Yang Y, Xie D, et al., 2019, Trabecular-like Ti-6Al- 4V scaffolds for orthopedic: fabrication by selective laser melting and in vitro biocompatibility. J Mater Sci Technol, 35:1284–1297. https://doi.org/10.1016/j.jmst.2019.01.012
- Li Y, 2014, Deformable Geometry Design with Controlled Mechanical Property Based on 3D Printing, University of Southern California, 162.
- Radman A, 2021, Combination of BESO and harmony search for topology optimization of microstructures for materials. Appl Math Model, 90:650–661. https://doi.org/10.1016/j.apm.2020.09.024
- Hutmacher DW, 2000, Scaffolds in tissue engineering bone and cartilage. Biomaterials, 21:2529–2543. https://doi.org/10.1016/s0142-9612(00)00121-6
- Yan QY, Cheng Y, Wang RR, et al., 2022, Recent advances in 3D porous MXenes: Structures, properties and applications. J Phys D-Appl Phys, 55:15. https://doi.org/10.1088/1361-6463/ac2db2
- Prananingrum W, Naito Y, Galli S, et al., 2016, Bone ingrowth of various porous titanium scaffolds produced by a moldless and space holder technique: an in vivo study in rabbits. Biomed Mater, 11:8. https://doi.org/10.1088/1748-6041/11/1/015012
- Babaie E, Bhaduri SB, 2018, Fabrication aspects of porous biomaterials in orthopedic applications: A review, ACS Biomater Sci Eng, 4:1–39. https://doi.org/10.1021/acsbiomaterials.7b00615
- Zheng YH, Han Q, Wang JC, et al., 2020, Promotion of osseointegration between implant and bone interface by titanium alloy porous scaffolds prepared by 3D printing. ACS Biomater Sci Eng, 6:5181–5190. https://doi.org/10.1021/acsbiomaterials.0c00662
- Zhang S, 2016, Foam metal research and application progress. Powder Metall Technol, 34:222–227.
- Qiu SW, Zhang XN, Hao QX, et al., 2015, Research progress on simulation modeling of metal foams. Rare Metal Mat Eng, 44:2670–2676.
- Gibson LJ, 2003, Cellular solids. MRS Bull, 28:270–271. https://doi.org/10.1557/mrs2003.79
- Gibson LJ, Ashby MF, 1997, Cellular Solids: Structure and Properties, Cambridge University Press, Cambridge, 387– 428.
- Mullen L, Stamp RC, Brooks WK, et al., 2009, Selective laser melting: A regular unit cell approach for the manufacture of porous, titanium, bone in-growth constructs, suitable for orthopedic applications. J Biomed Mater Res Part B, 89B:325–334. https://doi.org/10.1002/jbm.b.31219
- Craeghs T, Clijsters S, Yasa E, et al., 2011, Determination of geometrical factors in Layerwise laser melting using optical process monitoring. Opt Lasers Eng, 49:1440–1446. https://doi.org/10.1016/j.optlaseng.2011.06.016
- Wauthle R, Vrancken B, Beynaerts B, et al., 2015, Effects of build orientation and heat treatment on the microstructure and mechanical properties of selective laser melted Ti6Al4V lattice structures. Addit Manuf, 5:77–84. https://doi.org/10.1016/j.addma.2014.12.008
- Popovich A, Sufiiarov V, Borisov E, et al., 2015, Microstructure and mechanical properties of Ti-6Al-4V manufactured by SLM. Key Eng Mater (Switzerland), 651– 653: 677–682. https://doi.org/10.4028/www.scientific.net/KEM.651- 653.677
- Sevilla P, Aparicio C, Planell JA, et al., 2007, Comparison of the mechanical properties between tantalum and nickel-titanium foams implant materials for bone ingrowth applications. J Alloy Compd, 439:67–73. https://doi.org/10.1016/j.jallcom.2006.08.069
- Morgan EF, Bayraktar HH, Keaveny TM, 2003, Trabecular bone modulus-density relationships depend on anatomic site. J Biomech, 36:897–904. https://doi.org/10.1016/s0021-9290(03)00071-x