3D printing of biomaterials for vascularized and innervated tissue regeneration
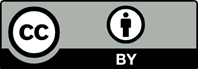
Neurovascular networks play significant roles in the metabolism and regeneration of many tissues and organs in the human body. Blood vessels can transport sufficient oxygen, nutrients, and biological factors, while nerve fibers transmit excitation signals to targeted cells. However, traditional scaffolds cannot satisfy the requirement of stimulating angiogenesis and innervation in a timely manner due to the complexity of host neurovascular networks. Three-dimensional (3D) printing, as a versatile and favorable technique, provides an effective approach to fabricating biological scaffolds with biomimetic architectures and multimaterial compositions, which are capable of regulating multiple cell behaviors. This review paper presents a summary of the current progress in 3D-printed biomaterials for vascularized and innervated tissue regeneration by presenting skin, bone, and skeletal muscle tissues as an example. In addition, we highlight the crucial roles of blood vessels and nerve fibers in the process of tissue regeneration and discuss the future perspectives for engineering novel biomaterials. It is expected that 3D-printed biomaterials with angiogenesis and innervation properties can not only recapitulate the physiological microenvironment of damaged tissues but also rapidly integrate with host neurovascular networks, resulting in accelerated functional tissue regeneration.
1. Marrella A, Lee TY, Lee DH, et al., 2018, Engineering vascularized and innervated bone biomaterials for improved skeletal tissue regeneration. Mater Today, 21(4):362–376. https://doi.org/10.1016/j.mattod.2017.10.005
2. Eugenis I, Wu D, Rando TA, 2021, Cells, scaffolds, and bioactive factors: Engineering strategies for improving regeneration following volumetric muscle loss. Biomaterials, 278:121173. https://doi.org/10.1016/j.biomaterials.2021.121173
3. Weng T, Wu P, Zhang W, et al., 2020, Regeneration of skin appendages and nerves: Current status and further challenges. J Trans Med, 18(1):1–17. https://doi.org/10.1186/s12967-020-02248-5
4. Wan Q-Q, Qin W-P, Shen M-J, et al., 2020, Simultaneous regeneration of bone and nerves through materials and architectural design: Are we there yet? Adv Funct Mater, 30(48):2003542. https://doi.org/10.1002/adfm.202003542
5. Ma J, Wu C, 2022, Bioactive inorganic particles-based biomaterials for skin tissue engineering. Exploration, 2(5):20210083. https://doi.org/10.1002/EXP.20210083
6. Gilbert-Honick J, Grayson W, 2020, Vascularized and innervated skeletal muscle tissue engineering. Adv Healthc Mater, 9(1):1900626. https://doi.org/10.1002/adhm.201900626
7. Beachley VZ, Wolf MT, Sadtler K, et al., 2015, Tissue matrix arrays for high-throughput screening and systems analysis of cell function. Nat Methods, 12(12): 1197-+. https://doi.org/10.1038/nmeth.3619
8. Marenzana M, Arnett TR, 2013, The key role of the blood supply to bone. Bone Res, 1:203–215. https://doi.org/10.4248/br201303001
9. Das S, Gordian-Velez WJ, Ledebur HC, et al., 2020, Innervation: The missing link for biofabricated tissues and organs. NPJ Regen Med, 5(1):1–17. https://doi.org/10.1038/s41536-020-0096-1
10. Su Y-W, Zhou X-F, Foster BK, et al., 2018, Roles of neurotrophins in skeletal tissue formation and healing. J Cell Physiol, 233(3): 2133–2145. https://doi.org/10.1002/jcp.25936
11. Chen W, Mao C, Zhuo L, et al., 2015, Beta-nerve growth factor promotes neurogenesis and angiogenesis during the repair of bone defects. Neural Regen Res, 10(7):1159–1165. https://doi.org/10.4103/1673-5374.160114
12. Mi J, Xu J, Yao H, et al., 2021, Calcitonin gene-related peptide enhances distraction osteogenesis by increasing angiogenesis. Tissue Eng Part A, 27(1-2):87-102. https://doi.org/10.1089/ten.tea.2020.0009
13. Guo Y, Chen H, Jiang Y, et al., 2020, CGRP regulates the dysfunction of peri-implant angiogenesis and osseointegration in streptozotocin-induced diabetic rats. Bone, 139:115464. https://doi.org/10.1016/j.bone.2020.115464
14. Bittner SM, Guo JL, Melchiorri A, et al., 2018, Three-dimensional printing of multilayered tissue engineering scaffolds. Mater Today, 21(8):861–874. https://doi.org/10.1016/j.mattod.2018.02.006
15. Heinrich MA, Liu W, Jimenez A, et al., 2019, 3D bioprinting: From benches to translational applications. Small, 15(23):1805510. https://doi.org/10.1002/smll.201805510
16. Li Q, Wang Z, 2013, Influence of mesenchymal stem cells with endothelial progenitor cells in co-culture on osteogenesis and angiogenesis: An in vitro study. Arch Med Res, 44(7):504–513. https://doi.org/10.1016/j.arcmed.2013.09.009
17. Street J, Bao M, deGuzman L, et al., 2002, Vascular endothelial growth factor stimulates bone repair by promoting angiogenesis and bone turnover. Proc Natl Acad Sci USA, 99(15):9656–9661. https://doi.org/10.1073/pnas.152324099
18. Laschke MW, Harder Y, Amon M, et al., 2006, Angiogenesis in tissue engineering: Breathing life into constructed tissue substitutes. Tissue Eng, 12(8):2093–2104. https://doi.org/10.1089/ten.2006.12.2093
19. Blais M, Parenteau-Bareil R, Cadau S, et al., 2013, Concise review: Tissue-engineered skin and nerve regeneration in burn treatment. Stem Cells Transl Med, 2(7):545–551. http://dx.doi.org/10.5966/sctm.2012-0181
20. Koons GL, Diba M, Mikos AG, 2020, Materials design for bone-tissue engineering. Nat Rev Mater, 5(8):584–603. https://doi.org/10.1038/s41578-020-0204-2
21. Ashrafi M, Baguneid M, Bayat A, 2016, The role of neuromediators and innervation in cutaneous wound healing. Acta Dermato-Venereol, 96(5):587-+. https://doi.org/10.2340/00015555-2321
22. Lumpkin EA, Caterina MJ, 2007, Mechanisms of sensory transduction in the skin. Nature, 445(7130):858–865. https://doi.org/10.1038/nature05662
23. Cheret J, Lebonvallet N, Buhe V, et al., 2014, Influence of sensory neuropeptides on human cutaneous wound healing process. J Dermatol Sci, 74(3):193–203. http://dx.doi.org/10.1016/j.jdermsci.2014.02.001
24. Theocharidis G, Veves A, 2020, Autonomic nerve dysfunction and impaired diabetic wound healing: The role of neuropeptides. Autonomic Neurosci Basic Clin, 223:102610. https://doi.org/10.1016/j.autneu.2019.102610
25. Blais M, Mottier L, Germain M-A, et al., 2014, Sensory neurons accelerate skin reepithelialization via substance P in an innervated tissue-engineered wound healing model. Tissue Eng Part A, 20(15-16):2180–2188. https://doi.org/10.1089/ten.tea.2013.0535
26. Sayilekshmy M, Hansen RB, Delaisse J-M, et al., 2019, Innervation is higher above bone remodeling surfaces and in cortical pores in human bone: Lessons from patients with primary hyperparathyroidism. Sci Rep, 9:5361. https://doi.org/10.1038/s41598-019-41779-w
27. Hohmann EL, Elde RP, Rysavy JA, et al., 1986, Innervation of periosteum and bone by sympathetic vasoactive intestinal peptide-containing nerve fibers. Science, 232(4752):868–871. https://doi.org/10.1126/science.3518059
28. Wan Q-Q, Qin W-P, Ma Y-X, et al., 2021, Crosstalk between bone and nerves within bone. Adv Sci, 8(7):2003390. https://doi.org/10.1002/advs.202003390
29. Wang X-D, Li S-Y, Zhang S-J, et al., 2020, The neural system regulates bone homeostasis via mesenchymal stem cells: a translational approach. Theranostics, 10(11):4839–4850. https://doi.org/10.7150/thno.43771
30. He H, Chai J, Zhang S, et al., 2016, CGRP may regulate bone metabolism through stimulating osteoblast differentiation and inhibiting osteoclast formation. Mol Med Rep, 13(5):3977–3984. https://doi.org/10.3892/mmr.2016.5023
31. Graessel S, 2014, The role of peripheral nerve fibers and their neurotransmitters in cartilage and bone physiology and pathophysiology. Arthritis Res Ther, 16(6):485. https://doi.org/10.1186/s13075-014-0485-1
32. Moore RE, Smith CK, Bailey CS, et al., 1993, Characterization of beta-adrenergic receptors on rat and human osteoblast-like cells and demonstration that beta-receptor agonists can stimulate bone resorption in organ culture. Bone Miner, 23(3):301–315. https://doi.org/10.1016/S0169-6009(08)80105-5
33. Katayama Y, Battista M, Kao W-M, et al., 2006, Signals from the sympathetic nervous system regulate hematopoietic stem cell egress from bone marrow. Cell, 124(2):407–421. https://doi.org/10.1016/j.cell.2005.10.041
34. Elefteriou F, 2018, Impact of the autonomic nervous system on the skeleton. Physiol Rev, 98(3):1083–1112. https://doi.org/10.1152/physrev.00014.2017
35. Sun S, Diggins NH, Gunderson ZJ, et al., 2020, No pain, no gain? The effects of pain-promoting neuropeptides and neurotrophins on fracture healing. Bone, 131:115109. https://doi.org/10.1016/j.bone.2019.115109
36. Meyers CA, Lee S, Sono T, et al., 2020, A neurotrophic mechanism directs sensory nerve transit in cranial bone. Cell Rep, 31(8):107696. https://doi.org/10.1016/j.celrep.2020.107696
37. Liu Q, Lei L, Yu T, et al., 2018, Effect of brain-derived neurotrophic factor on the neurogenesis and osteogenesis in bone engineering. Tissue Eng Part A, 24(15-16):1283–1292. https://doi.org/10.1089/ten.tea.2017.0462
38. Ai L-S, Sun C-Y, Zhang L, et al., 2012, Inhibition of BDNF in multiple myeloma blocks osteoclastogenesis via down-regulated stroma-derived RANKL expression both in vitro and in vivo. PLoS One, 7(10):e46287. https://doi.org/10.1371/journal.pone.0046287
39. Cai X-x, Luo E, Yuan Q, 2010, Interaction between schwann cells and osteoblasts in vitro. Int J Oral Sci, 2(2):74–81. https://doi.org/10.4248/ijos10039
40. Jones RE, Salhotra A, Robertson KS, et al., 2019, Skeletal stem cell-schwann cell circuitry in mandibular repair. Cell Rep, 28(11):2757–2766.e5. https://doi.org/10.1016/j.celrep.2019.08.021
41. Samandari M, Quint J, Rodriguez-delaRosa A, et al., 2022, Bioinks and bioprinting strategies for skeletal muscle tissue engineering. Adv Mater, 34(12):21105883. https://doi.org/10.1002/adma.202105883
42. Gilbert-Honick J, Iyer SR, Somers SM, et al., 2020, Engineering 3D skeletal muscle primed for neuromuscular regeneration following volumetric muscle loss. Biomaterials, 255:120154. https://doi.org/10.1016/j.biomaterials.2020.120154
43. Raffa P, Easler M, Urciuolo A, 2022, Three-dimensional in vitro models of neuromuscular tissue. Neural Regen Res, 17(4):759–766. https://doi.org/10.4103/1673-5374.322447
44. Carmeliet P, 2003, Blood vessels and nerves: Common signals, pathways and diseases. Nat Rev Genet, 4(9):710–720. https://doi.org/10.1038/nrg1158
45. Carmeliet P, Tessier-Lavigne M, 2005, Common mechanisms of nerve and blood vessel wiring. Nature, 436(7048): 193–200. https://doi.org/10.1038/nature03875
46. Morotti M, Vincent K, Brawn J, et al., 2014, Peripheral changes in endometriosis-associated pain. Hum Reprod Update, 20(5):717–736. https://doi.org/10.1093/humupd/dmu021
47. Raab S, Plate KH, 2007, Different networks, common growth factors: Shared growth factors and receptors of the vascular and the nervous system. Acta Neuropathol, 113(6):607–626. https://doi.org/10.1007/s00401-007-0228-3
48. Troullinaki M, Alexaki V-I, Mitroulis I, et al., 2019, Nerve growth factor regulates endothelial cell survival and pathological retinal angiogenesis. J Cell Mol Med, 23(4):2362–2371. https://doi.org/10.1111/jcmm.14002
49. Emanueli C, Salis MB, Pinna A, et al., 2002, Nerve growth factor promotes angiogenesis and arteriogenesis in ischemic hindlimbs. Circulation, 106(17):2257–2262. https://doi.org/10.1161/01.CIR.0000033971.56802.C5
50. Hecking I, Stegemann LN, Theis V, et al., 2022, Neuroprotective effects of VEGF in the enteric nervous system. Int J Mol Sci, 23(12):6756. https://doi.org/10.3390/ijms23126756
51. Huang Y, Zhang L, Ji Y, et al., 2023, A non-invasive smart scaffold for bone repair and monitoring. Bioact Mater, 19:499–510. https://doi.org/10.1016/j.bioactmat.2022.04.034
52. Murphy SV, De Coppi P, Atala A, 2020, Opportunities and challenges of translational 3D bioprinting. Nat Biomed Eng, 4(4):370–380. https://doi.org/10.1038/s41551-019-0471-7
53. Cheng L, Cai Z, Ye T, et al., 2020, Injectable polypeptide-protein hydrogels for promoting infected wound healing. Adv Funct Mater, 30(25):2001196. https://doi.org/10.1002/adfm.202001196
54. Yang H, Lai C, Xuan C, et al., 2020, Integrin-binding pro-survival peptide engineered silk fibroin nanosheets for diabetic wound healing and skin regeneration. Chem Eng J, 398:125617. https://doi.org/10.1016/j.cej.2020.125617
55. Yao S, Wang Y, Chi J, et al., 2022, Porous MOF microneedle array patch with photothermal responsive nitric oxide delivery for wound healing. Adv Sci, 9(3):2103449. https://doi.org/10.1002/advs.202103449
56. Chen H, Guo Y, Zhang Z, et al., 2022, Symbiotic algae-bacteria dressing for producing hydrogen to accelerate diabetic wound healing. Nano Lett, 22(1):229–237. https://doi.org/10.1021/acs.nanolett.1c03693
57. Yao S, Chi J, Wang Y, et al., 2021, Zn-MOF encapsulated antibacterial and degradable microneedles array for promoting wound healing. Adv Healthc Mater, 10(12):2100056. https://doi.org/10.1002/adhm.202100056
58. Yin M, Wu J, Deng M, et al., 2021, Multifunctional magnesium organic framework-based microneedle patch for accelerating diabetic wound healing. Acs Nano, 15(11):17842–17853. https://doi.org/10.1021/acsnano.1c06036
59. Deng Z, Li M, Hu Y, et al., 2021, Injectable biomimetic hydrogels encapsulating Gold/metal-organic frameworks nanocomposites for enhanced antibacterial and wound healing activity under visible light actuation. Chem Eng J, 420:129668. https://doi.org/10.1016/j.cej.2021.129668
60. Xiao J, Zhu Y, Huddleston S, et al., 2018, Copper metal-organic framework nanoparticles stabilized with folic acid improve wound healing in diabetes. ACS Nano, 12(2):1023–1032. https://doi.org/10.1021/acsnano.7b01850
61. Zhao X, Wu H, Guo B, et al., 2017, Antibacterial anti-oxidant electroactive injectable hydrogel as self-healing wound dressing with hemostasis and adhesiveness for cutaneous wound healing. Biomaterials, 122:34–47. https://doi.org/10.1016/j.biomaterials.2017.01.011
62. Ibanez RIR, do Amaral RJFC, Simpson CR, et al., 2022, 3D printed scaffolds incorporated with platelet-rich plasma show enhanced angiogenic potential while not inducing fibrosis. Adv Funct Mater, 32(10):2109915. https://doi.org/10.1002/adfm.202109915
63. Wang X, Yu Y, Yang C, et al., 2022, Dynamically responsive scaffolds from microfluidic 3D printing for skin flap regeneration. Adv Sci, 9(22):2201155. https://doi.org/10.1002/advs.202201155
64. Alizadehgiashi M, Nemr CR, Chekini M, et al., 2021, Multifunctional 3D-printed wound dressings. ACS Nano, 15(7):12375–12387. https://doi.org/10.1021/acsnano.1c04499
65. Singh S, Choudhury D, Yu F, et al., 2020, In situ bioprinting— Bioprinting from benchside to bedside? Acta Biomater, 101:14-25. https://doi.org/10.1016/j.actbio.2019.08.045
66. Kong L, Wu Z, Zhao H, et al., 2018, Bioactive injectable hydrogels containing desferrioxamine and bioglass for diabetic wound healing. ACS Appl Mater Interface, 10(36):30103–30114. https://doi.org/10.1021/acsami.8b09191
67. Albanna M, Binder KW, Murphy SV, et al., 2019, In situ bioprinting of autologous skin cells accelerates wound healing of extensive excisional full-thickness wounds. Sci Rep, 9:1856. https://doi.org/10.1038/s41598-018-38366-w
68. Hakimi N, Cheng R, Leng L, et al., 2018, Handheld skin printer: In situ formation of planar biomaterials and tissues. Lab Chip, 18(10):1440–1451. https://doi.org/10.1039/c7lc01236e
69. Nuutila K, Samandari M, Endo Y, et al., 2022, In vivo printing of growth factor-eluting adhesive scaffolds improves wound healing. Bioact Mater, 8:296–308. https://doi.org/10.1016/j.bioactmat.2021.06.030
70. Phan J, Kumar P, Hao D, et al., 2018, Engineering mesenchymal stem cells to improve their exosome efficacy and yield for cell-free therapy. J Extracell Vesicles, 7(1):1522236. https://doi.org/10.1080/20013078.2018.1522236
71. Hu Y, Tao R, Chen L, et al., 2021, Exosomes derived from pioglitazone-pretreated MSCs accelerate diabetic wound healing through enhancing angiogenesis. J Nanobiotechnol, 19(1):150. https://doi.org/10.1186/s12951-021-00894-5
72. Hu Y, Wu B, Xiong Y, et al., 2021, Cryogenic 3D printed hydrogel scaffolds loading exosomes accelerate diabetic wound healing. Chem Eng J, 426:130634. https://doi.org/10.1016/j.cej.2021.130634
73. Thapa RK, Diep DB, Tonnesen HH, 2020, Topical antimicrobial peptide formulations for wound healing: Current developments and future prospects. Acta Biomater, 103:52–67. https://doi.org/10.1016/j.actbio.2019.12.025
74. Chu B, He J-m, Wang Z, et al., 2021, Proangiogenic peptide nanofiber hydrogel/3D printed scaffold for dermal regeneration. Chem Eng J, 424:128146. https://doi.org/10.1016/j.cej.2020.128146
75. Yu Q, Han Y, Tian T, et al., 2019, Chinese sesame stick-inspired nano-fibrous scaffolds for tumor therapy and skin tissue reconstruction. Biomaterials, 194:25–35. https://doi.org/10.1016/j.biomaterials.2018.12.012
76. Yu Q, Han Y, Wang X, et al., 2018, Copper silicate hollow microspheres-incorporated scaffolds for chemo-photothermal therapy of melanoma and tissue healing. ACS Nano, 12(3):2695–2707. https://doi.org/10.1021/acsnano.7b08928
77. Wang X, Xue J, Ma B, et al., 2020, Black bioceramics: Combining regeneration with therapy. Adv Mater, 32(48):2005140. https://doi.org/10.1002/adma.202005140
78. Xu C, Xu Y, Yang M, et al., 2020, Black-phosphorus-incorporated hydrogel as a conductive and biodegradable platform for enhancement of the neural differentiation of mesenchymal stem cells. Adv Funct Mater, 30(39):2000177. https://doi.org/10.1002/adfm.202000177
79. Saghiri MA, Asatourian A, Orangi J, et al., 2015, Functional role of inorganic trace elements in angiogenesis-Part I: N, Fe, Se, P, Au, and Ca. Crit Rev Oncol Hematol, 96(1): 129–142. https://dx.doi.org/10.1016/j.critrevonc.2015.05.010
80. Ma W, Ma H, Qiu P, et al., 2021, Sprayable beta-FeSi2 composite hydrogel for portable skin tumor treatment and wound healing. Biomaterials, 279:121225. https://doi.org/10.1016/j.biomaterials.2021.121225
81. Sheng L, Zhang Z, Zhang Y, et al., 2021, A novel “hot spring”- mimetic hydrogel with excellent angiogenic properties for chronic wound healing. Biomaterials, 264:120414. https://doi.org/10.1016/j.biomaterials.2020.120414
82. Ma J, Wu J, Zhang H, et al., 2022, 3D printing of diatomite incorporated composite scaffolds for skin repair of deep burn wounds. Int J Bioprint, 8(3):163–175. https://doi.org/10.18063/ijb.v8i3.580
83. Murphy SV, Atala A, 2014, 3D bioprinting of tissues and organs. Nat Biotech, 32(8):773–785. https://doi.org/10.1038/nbt.2958
84. Zhou F, Hong Y, Liang R, et al., 2020, Rapid printing of bio-inspired 3D tissue constructs for skin regeneration. Biomaterials, 258:120287. 10.1016/j.biomaterials.2020.120287
85. Ma J, Qin C, Wu J, et al., 2021, 3D printing of strontium silicate microcylinder-containing multicellular biomaterial inks for vascularized skin regeneration. Adv Healthc Mater, 10(16):2100523. https://doi.org/10.1002/adhm.202100523
86. Wu J, Qin C, Ma J, et al., 2021, An immunomodulatory bioink with hollow manganese silicate nanospheres for angiogenesis. Appl Mater Today, 23:101015. https://doi.org/10.1016/j.apmt.2021.101015
87. Fan L, Xiao C, Guan P, et al., 2022, Extracellular matrix-based conductive interpenetrating network hydrogels with enhanced neurovascular regeneration properties for diabetic wounds repair. Adv Healthc Mater, 11(1):2101556. https://doi.org/10.1002/adhm.202101556
88. Wang J, Lin J, Chen L, et al., 2022, Endogenous electric-field-coupled electrospun short fiber via collecting wound exudation. Adv Mater, 34(9):2108325. https://doi.org/10.1002/adma.202108325
89. Sebastian A, Volk SW, Halai P, et al., 2017, Enhanced neurogenic biomarker expression and reinnervation in human acute skin wounds treated by electrical stimulation. J Investig Dermatol, 137(3):737–747. https://doi.org/10.1016/j.jid.2016.09.038
90. Emmerson E, 2017, Efficient healing takes some nerve: Electrical stimulation enhances innervation in cutaneous human wounds. J Investig Dermatol, 137(3):543–545. https://doi.org/10.1016/j.jid.2016.10.018
91. Tan M-h, Xu X-h, Yuan T-j, et al., 2022, Self-powered smart patch promotes skin nerve regeneration and sensation restoration by delivering biological-electrical signals in program. Biomaterials, 283:121413. https://doi.org/10.1016/j.biomaterials.2022.121413
92. Peng L-H, Xu X-H, Huang Y-F, et al., 2020, Self-adaptive all-in-one delivery chip for rapid skin nerves regeneration by endogenous mesenchymal stem cells. Adv Funct Mater, 30(40):2001751. https://doi.org/10.1002/adfm.202001751
93. Qian Z, Wang H, Bai Y, et al., 2020, Improving chronic diabetic wound healing through an injectable and self-healing hydrogel with platelet-rich plasma release. ACS Appl Mater Interface, 12(50):55659-55674. https://doi.org/10.1021/acsami.0c17142
94. Xu X-H, Yuan T-J, Dad HA, et al., 2021, Plant exosomes as novel nanoplatforms for microRNA transfer stimulate neural differentiation of stem cells in vitro and in vivo. Nano Lett, 21(19):8151–8159. https://doi.org/10.1021/acs.nanolett.1c02530
95. Brokesh AM, Gaharwar AK, 2020, Inorganic biomaterials for regenerative medicine. ACS Appl Mater Interface, 12(5):5319–5344. https://doi.org/10.1021/acsami.9b17801
96. Sun L, Wang M, Chen S, et al., 2019, Molecularly engineered metal-based bioactive soft materials—Neuroactive magnesium ion/polymer hybrids. Acta Biomater, 85: 310–319. https://doi.org/10.1016/j.actbio.2018.12.040
97. Zhang H, Ma W, Ma H, et al., 2022, Spindle-like zinc silicate nanoparticles accelerating innervated and vascularized skin burn wound healing. Adv Healthc Mater, 11(10):2102359. https://doi.org/10.1002/adhm.202102359
98. Li T, Zhai D, Ma B, et al., 2019, 3D printing of hot dog-like biomaterials with hierarchical architecture and distinct bioactivity. Adv Sci, 6(19):1901146. https://doi.org/10.1002/advs.201901146
99. Li Y, Xu J, Mi J, et al., 2021, Biodegradable magnesium combined with distraction osteogenesis synergistically stimulates bone tissue regeneration via CGRP-FAK-VEGF signaling axis. Biomaterials, 275:120984. https://doi.org/10.1016/j.biomaterials.2021.120984
100. Li T, Chang J, Zhu Y, et al., 2020, 3D printing of bioinspired biomaterials for tissue regeneration. Adv Healthc Mater, 9(23):2000208. https://doi.org/10.1002/adhm.202000208
101. Zhang W, Feng C, Yang G, et al., 2017, 3D-printed scaffolds with synergistic effect of hollow-pipe structure and bioactive ions for vascularized bone regeneration. Biomaterials, 135:85–95. https://doi.org/10.1016/j.biomaterials.2017.05.005
102. Chung JJ, Yoo J, Sum BST, et al., 2021, 3D printed porous methacrylate/silica hybrid scaffold for bone substitution. Adv Healthc Mater, 10(12):2100117. https://doi.org/10.1002/adhm.202100117
103. Wang C, Lai J, Li K, et al., 2021, Cryogenic 3D printing of dual-delivery scaffolds for improved bone regeneration with enhanced vascularization. Bioact Mater, 6(1):137–145. https://doi.org/10.1016/j.bioactmat.2020.07.007
104. Byambaa B, Annabi N, Yue K, et al., 2017, Bioprinted osteogenic and vasculogenic patterns for engineering 3D bone tissue. Adv Healthc Mater, 6(16):1700015. https://doi.org/10.1002/adhm.201700015
105. Piard C, Baker H, Kamalitdinov T, et al., 2019, Bioprinted osteon-like scaffolds enhance in vivo neovascularization. Biofabrication, 11(2):025013. https://doi.org/10.1088/1758-5090/ab078a
106. Sun X, Ma Z, Zhao X, et al., 2021, Three-dimensional bioprinting of multicell-laden scaffolds containing bone morphogenic protein-4 for promoting M2 macrophage polarization and accelerating bone defect repair in diabetes mellitus. Bioact Mater, 6(3):757–769. https://doi.org/10.1016/j.bioactmat.2020.08.030
107. Wan Z, Zhang P, Liu Y, et al., 2020, Four-dimensional bioprinting: Current developments and applications in bone tissue engineering. Acta Biomater, 101:26–42. https://doi.org/10.1016/j.actbio.2019.10.038
108. Feng C, Zhang W, Deng C, et al., 2017, 3D printing of lotus root-like biomimetic materials for cell delivery and tissue regeneration. Adv Sci, 4(12):1700401. https://doi.org/10.1002/advs.201700401
109. Hann SY, Cui H, Esworthy T, et al., 2021, Dual 3D printing for vascularized bone tissue regeneration. Acta Biomater, 123:263–274. https://doi.org/10.1016/j.actbio.2021.01.012
110. Wang X, Yu Y, Yang C, et al., 2021, Microfluidic 3D printing responsive scaffolds with biomimetic enrichment channels for bone regeneration. Adv Funct Mater, 31(40):2105190. https://doi.org/10.1002/adfm.202105190
111. Zhang M, Lin R, Wang X, et al., 2020, 3D printing of Haversian bone-mimicking scaffolds for multicellular delivery in bone regeneration. Sci Adv, 6(12):eaaz6725. https://doi.org/10.1126/sciadv.aaz6725
112. Wang L, Hu P, Jiang H, et al., 2022, Mild hyperthermia-mediated osteogenesis and angiogenesis play a critical role in magnetothermal composite-induced bone regeneration. Nano Today, 43:101401. https://doi.org/10.1016/j.nantod.2022.101401
113. Yu X, Wang X, Li D, et al., 2022, Mechanically reinforced injectable bioactive nanocomposite hydrogels for in-situ bone regeneration. Chem Eng J, 433:132799. https://doi.org/10.1016/j.cej.2021.132799
114. Zhu D, Lu B, Yang Q, et al., 2021, Lanthanum-doped mesoporous bioglasses/chitosan composite scaffolds enhance synchronous osteogenesis and angiogenesis for augmented osseous regeneration. Chem Eng J, 405:127077. https://doi.org/10.1016/j.cej.2020.127077
115. Yin J, Pan S, Guo X, et al., 2021, Nb2C MXene-functionalized scaffolds enables osteosarcoma phototherapy and angiogenesis/osteogenesis of bone defects. Nano-Micro Lett, 13(1): 30. https://doi.org/10.1007/s40820-020-00547-6
116. Han X, Sun M, Chen B, et al., 2021, Lotus seedpod-inspired internal vascularized 3D printed scaffold for bone tissue repair. Bioact Mater, 6(6):1639–1652. https://doi.org/10.1016/j.bioactmat.2020.11.019
117. Gu J, Zhang Q, Geng M, et al., 2021, Construction of nanofibrous scaffolds with interconnected perfusable microchannel networks for engineering of vascularized bone tissue. Bioact Mater, 6(10):3254–3268. https://doi.org/10.1016/j.bioactmat.2021.02.033
118. Ha Y, Ma X, Li S, et al., 2022, Bone microenvironment-mimetic scaffolds with hierarchical microstructure for enhanced vascularization and bone regeneration. Adv Funct Mater, 32(20):2200011. https://doi.org/10.1002/adfm.202200011
119. Yang C, Zheng Z, Younis MR, et al., 2021, 3D printed enzyme-functionalized scaffold facilitates diabetic bone regeneration. Adv Funct Mater, 31(20):2101372. https://doi.org/10.1002/adfm.202101372
120. Li W, Miao W, Liu Y, et al., 2022, Bioprinted constructs that mimic the ossification center microenvironment for targeted innervation in bone regeneration. Adv Funct Mater, 32(9):2109871. https://doi.org/10.1002/adfm.202109871
121. Fitzpatrick V, Martin-Moldes Z, Deck A, et al., 2021, Functionalized 3D-printed silk-hydroxyapatite scaffolds for enhanced bone regeneration with innervation and vascularization. Biomaterials, 276:120995. https://doi.org/10.1016/j.biomaterials.2021.120995
122. Zhang Y, Xu J, Ruan YC, et al., 2016, Implant-derived magnesium induces local neuronal production of CGRP to improve bone-fracture healing in rats. Nat Med, 22(10):1160–1169. https://doi.org/10.1038/nm.4162
123. Ma Y-X, Jiao K, Wan Q-Q, et al., 2022, Silicified collagen scaffold induces semaphorin 3A secretion by sensory nerves to improve in-situ bone regeneration. Bioact Mater, 9: 475–490. https://doi.org/10.1016/j.bioactmat.2021.07.016
124. Zhang M, Qin C, Wang Y, et al., 2022, 3D printing of tree-like scaffolds for innervated bone regeneration. Addit Manuf, 54:102721. https://doi.org/10.1016/j.addma.2022.102721
125. Zhang H, Qin C, Zhang M, et al., 2022, Calcium silicate nanowires-containing multicellular bioinks for 3D bioprinting of neural-bone constructs. Nano Today, 46:101584. https://doi.org/10.1016/j.nantod.2022.101584
126. Li T, Hou J, Wang L, et al., 2022, Bioprinted anisotropic scaffolds with fast stress relaxation bioink for engineering 3D skeletal muscle and repairing volumetric muscle loss. Acta Biomater, 156:21–36’. https://doi.org/10.1016/j.actbio.2022.08.037
127. Hwangbo H, Lee H, Jin E-J, et al., 2022, Bio-printing of aligned GelMa-based cell-laden structure for muscle tissue regeneration. Bioact Mater, 8:57–70. https://doi.org/10.1016/j.bioactmat.2021.06.031
128. Kim W, Lee H, Lee C K, et al., 2021, A bioprinting process supplemented with in situ electrical stimulation directly induces significant myotube formation and myogenesis. Adv Funct Mater, 31(51):2105170. https://doi.org/10.1002/adfm.202105170
129. Panayi AC, Smit L, Hays N, et al., 2020, A porous collagen-GAG scaffold promotes muscle regeneration following volumetric muscle loss injury. Wound Repair Regen, 28(1):61–74. https://doi.org/10.1111/wrr.12768
130. Zhang Z, Klausen LH, Chen M, et al., 2018, Electroactive scaffolds for neurogenesis and myogenesis: graphene-based nanomaterials. Small, 14(48):1801983. https://doi.org/10.1002/smll.201801983
131. Quint JP, Mostafavi A, Endo Y, et al., 2021, In vivo printing of nanoenabled scaffolds for the treatment of skeletal muscle injuries. Adv Healthc Mater, 10(10):2002152. https://doi.org/10.1002/adhm.202002152
132. Said SS, Yin H, Elfarnawany M, et al., 2019, Fortifying angiogenesis in ischemic muscle with FGF9-loaded electrospun poly(ester amide) fibers. Adv Healthc Mater, 8(8):1801294. https://doi.org/10.1002/adhm.201801294
133. Gholobova D, Terrie L, Gerard M, et al., 2020, Vascularization of tissue-engineered skeletal muscle constructs. Biomaterials, 235:119708. https://doi.org/10.1016/j.biomaterials.2019.119708
134. Choi Y-J, Jun Y-J, Kim D Y, et al., 2019, A 3D cell printed muscle construct with tissue-derived bioink for the treatment of volumetric muscle loss. Biomaterials, 206: 160–169. https://doi.org/10.1016/j.biomaterials.2019.03.036
135. Qazi TH, Mooney DJ, Pumberger M, et al., 2015, Biomaterials based strategies for skeletal muscle tissue engineering: Existing technologies and future trends. Biomaterials, 53:502–521. https://doi.org/10.1016/j.biomaterials.2015.02.110
136. Lee H, Kim W, Lee J, et al., 2021, Self-aligned myofibers in 3D bioprinted extracellular matrix-based construct accelerate skeletal muscle function restoration. Appl Phys Rev, 8(2):021405. https://doi.org/10.1063/5.0039639
137. Ostrovidov S, Salehi S, Costantini M, et al., 2019, 3D bioprinting in skeletal muscle tissue engineering. Small, 15(24):1805530. https://doi.org/10.1002/smll.201805530
138. Gao G, Cui X, 2016, Three-dimensional bioprinting in tissue engineering and regenerative medicine. Biotech Lett, 38(2):203–211. https://doi.org/10.1007/s10529-015-1975-1
139. Kim JH, Kim I, Seol Y-J, et al., 2020, Neural cell integration into 3D bioprinted skeletal muscle constructs accelerates restoration of muscle function. Nat Commun, 11(1):1025. https://doi.org/10.1038/s41467-020-14930-9
140. Mi J, Xu J-K, Yao Z, et al., 2022, Implantable electrical stimulation at dorsal root ganglions accelerates osteoporotic fracture healing via calcitonin gene-related peptide. Adv Sci, 9(1):2103005. https://doi.org/10.1002/advs.202103005