Hybrid biofabrication of neurosecretory structures as a model for neurosecretion
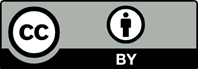
The present study aimed to combine extrusion-based three-dimensional (3D) bioprinting and polymer nanofiber electrospinning technology to fabricate tissuelike structures with neurosecretory function in vitro. Using neurosecretory cells as cell resources, sodium alginate/gelatin/fibrinogen as matrix, polylactic acid/gelatin electrospun nanofibers as diaphragm, and neurosecretory cells-loaded 3D hydrogel scaffolds were bioprinted and then covered with electrospun nanofibers layerby-layer. The morphology was observed by scanning electron microscopy and transmission electron microscopy (TEM), and the mechanical characteristics and cytotoxicity of the hybrid biofabricated scaffold structure were evaluated. The 3D-bioprinted tissue activity, including cell death and proliferation, was verified. Western blotting and ELISA experiments were used to confirm the cell phenotype and secretory function, while animal in vivo transplantation experiments confirmed the histocompatibility, inflammatory reaction, and tissue remodeling ability of the heterozygous tissue structures. Neurosecretory structures with 3D structures were successfully prepared by hybrid biofabrication in vitro. The mechanical strength of the composite biofabricated structures was significantly higher than that of the hydrogel system (P < 0.05). The survival rate of PC12 cells in the 3D-bioprinted model was 92.849 ± 2.995%. Hematoxylin and eosin-stained pathological sections showed that the cells grew in clumps, and there was no significant difference in the expression of MAP2 and tubulin-β between 3D organoids and PC12 cells. The results of ELISA showed that the PC12 cells in 3D structures retained the ability to continuously secrete noradrenaline and met-enkephalin, and the secretory vesicles around and within the cells could be observed by TEM. In in vivo transplantation, PC12 cells gathered and grew in clusters, maintained high activity, neovascularization, and tissue remodeling in 3D structures. The neurosecretory structures were biofabricated by 3D bioprinting and nanofiber electrospinning in vitro, which had high activity and neurosecretory function. In vivo transplantation of neurosecretory structures showed active proliferation of cells and potential for tissue remodeling. Our research provides a new method for biological manufacture of neurosecretory structures in vitro, which maintains neurosecretory function and lays the foundation for the clinical application of neuroendocrine tissues.
1. Yoo ES, Yu J, Sohn JW, 2021, Neuroendocrine control of appetite and metabolism. Exp Mol Med, 53: 505–516. https://doi.org/10.1038/s12276-021-00597-9
2. Téblick A, Gunst J, Langouche L, et al., 2022, Novel insights in endocrine and metabolic pathways in sepsis and gaps for future research. Clin Sci (Lond), 136: 861–878. https://doi.org/10.1042/CS20211003
3. Garrahy A, Sherlock M, Thompson CJ, 2017, Management of endocrine disease: Neuroendocrine surveillance and management of neurosurgical patients. Eur J Endocrinol, 176: R217–R233. https://doi.org/10.1530/EJE-16-0962
4. Schwerdtfeger LA, Tobet SA, 2019, From organotypic culture to body-on-a-chip: A neuroendocrine perspective. J Neuroendocrinol, 31: e12650. https://doi.org/10.1111/jne.12650
5. Jo Y, Hwang DG, Kim M, et al., 2022, Bioprinting-assisted tissue assembly to generate organ substitutes at scale. Trends Biotechnol, S0167–7799(22)00170-6. https://doi.org/10.1016/j.tibtech.2022.07.001
6. Jiang T, Munguia-Lopez JG, Flores-Torres S, et al., 2019, Extrusion bioprinting of soft materials: An emerging technique for biological model fabrication. Appl Phys Rev, 6: 011310. https://doi.org/10.1063/1.5059393
7. Zhuang P, Ng WL, An J, et al., 2019, Layer-by-layer ultraviolet assisted extrusion-based (UAE) bioprinting of hydrogel constructs with high aspect ratio for soft tissue engineering applications. PLoS One, 14: e0216776. https://doi.org/10.1371/journal.pone.0216776
8. Li X, Liu B, Pei B, et al., 2020, Inkjet bioprinting of biomaterials. Chem Rev, 120: 10793–10833. https://doi.org/10.1021/acs.chemrev.0c00008
9. Ng WL, Huang X, Shkolnikov V, et al., 2021, Controlling droplet impact velocity and droplet volume: Key factors to achieving high cell viability in sub-nanoliter droplet-based bioprinting. Int J Bioprint, 8: 424. https://doi.org/10.18063/ijb.v8i1.424
10. Ng WL, Lee JM, Zhou M, et al., 2020, Vat polymerization-based bioprinting-process, materials, applications and regulatory challenges. Biofabrication, 12: 022001. https://doi.org/10.1088/1758-5090/ab6034
11. Li W, Mille LS, Robledo JA, et al., 2020, Recent advances in formulating and processing biomaterial inks for vat polymerization-based 3d printing. Adv Healthc Mater, 9: e2000156.
12. Fonseca AC, Melchels FP, Ferreira MJ, et al., 2020, Emulating human tissues and organs: A bioprinting perspective toward personalized medicine. Chem Rev, 120: 11128–11174. doi: 10.1021/acs.chemrev.0c00342
13. Jain P, Kathuria H, Dubey N, et al., 2022, Advances in 3D bioprinting of tissues/organs for regenerative medicine and in-vitro models. Biomaterials, 287:121639. https://doi.org/10.1016/j.biomaterials.2022.121639
14. Cuvellier M, Rose S, Ezan F, et al., 2022, In vitro long term differentiation and functionality of three-dimensional bioprinted primary human hepatocytes: Application for in vivo engraftment. Biofabrication, 14:035021
https://doi.org/10.1088/1758-5090/ac7825
15. Bernal PN, Bouwmeester M, Madrid-Wolff J, et al., 2022, Volumetric bioprinting of organoids and optically tuned hydrogels to build liver-like metabolic biofactories. Adv Mater, 34(15):e2110054. https://doi.org/10.1002/adma.202110054
16. Ma L, Wu Y, Li Y, et al., 2020, Current advances on 3D-bioprinted liver tissue models. Adv Healthc Mater, 9: e2001517. https://doi.org/10.1002/adhm.202001517
17. Lawlor KT, Vanslambrouck JM, Higgins JW, et al., 2021, Cellular extrusion bioprinting improves kidney organoid reproducibility and conformation. Nat Mater, 20(2):260–271. https://doi.org/10.1038/s41563-020-00853-9
18. Ahrens JH, Uzel SG, Skylar-Scott M, et al., 2022, Programming cellular alignment in engineered cardiac tissue via bioprinting anisotropic organ building blocks. Adv Mater, 34: e2200217. https://doi.org/10.1002/dma.202200217
19. Li YE, Jodat YA, Samanipour R, et al., 2020, Toward a neurospheroid niche model: Optimizing embedded 3D bioprinting for fabrication of neurospheroid brain-like co-culture constructs. Biofabrication, 13: 015014. https://doi/.org/10.1088/1758-5090/abc1be
20. Bolívar-Monsalve EJ, Ceballos-González CF, Chávez- Madero C, et al., 2022, One-Step bioprinting of multi-channel hydrogel filaments using chaotic advection: Fabrication of pre-vascularized muscle-like tissues. Adv
Healthc Mater, 2200448: e2200448. https://doi.org/10.1002/adhm.202200448
21. Wu T, Gao YY, Su J, et al., 2022, Three-dimensional bioprinting of artificial ovaries by an extrusion-based method using gelatin-methacryloyl bioink. Climacteric, 25: 170–178. https://doi.org/10.1080/13697137.2021.1921726.
22. Bulanova EA, Koudan EV, Degosserie J, et al., 2017, Bioprinting of a functional vascularized mouse thyroid gland construct. Biofabrication, 9: 034105. https://doi.org/10.1088/1758-5090/aa7fdd
23. Yu HW, Kim BS, Lee JY, et al., 2021, Tissue printing for engineering transplantable human parathyroid patch to improve parathyroid engraftment, integration, and hormone secretion in vivo. Biofabrication, 13: 035033. https://doi.org/10.1088/1758-5090/abf740
24. Zubizarreta ME, Xiao S, 2020, Bioengineering models of female reproduction. Biodes Manuf, 3: 237–251. https://doi.org/10.1007/s42242-020-00082-8
25. Diao J, Zhang C, Zhang D, et al., 2019, Role and mechanisms of a three-dimensional bioprinted microtissue model in promoting proliferation and invasion of growth-hormone-secreting pituitary adenoma cells. Biofabrication, 11: 025006. https://doi.org/10.1088/1758-5090/aaf7ea
26. Castilho M, de Ruijter M, Beirne S, et al., 2020, Multitechnology biofabrication: A new approach for the manufacturing of functional tissue structures? Trends Biotechnol, 38: 1316–1328. https://doi.org/10.1016/j.tibtech.2020.04.014
27. Thakor J, Ahadian S, Niakan A, et al., 2020, Engineered hydrogels for brain tumor culture and therapy. Biodes Manuf, 3: 203–226. https://doi.org/10.1007/s42242-020-00084-6
28. Xu T, Binder KW, Albanna MZ, et al., 2013, Hybrid printing of mechanically and biologically improved constructs for cartilage tissue engineering applications. Biofabrication, 5: 015001. https://doi.org/10.1088/1758-5082/5/1/015001
29. Hwang DG, Jo Y, Kim M, et al., 2021, A 3D bioprinted hybrid encapsulation system for delivery of human pluripotent stem cell-derived pancreatic islet-like aggregates. Biofabrication, 14: 014101. https://doi.org/10.1088/1758-5090/ac23ac
30. Yoon Y, Kim CH, Lee JE, et al., 2019, 3D bioprinted complex constructs reinforced by hybrid multilayers of electrospun nanofiber sheets. Biofabrication, 11: 025015. https://doi.org/10.1088/1758-5090/ab08c2
31. Heydari Z, Moeinvaziri F, Agarwal T, et al., 2021, Organoids: A novel modality in disease modeling. Biodes Manuf, 4: 689–716. https://doi.org/10.1007/s42242-021-00150-7
32. Chen H, Wu Z, Gong Z, et al., 2022, Acoustic bioprinting of patient-derived organoids for predicting cancer therapy responses. Adv Healthc Mater, 11: e2102784. https://doi.org/10.1002/adhm.202102784
33. Dai X, Shao Y, Tian X, et al., 2022, Fusion between glioma stem cells and mesenchymal stem cells promotes malignant progression in 3D-bioprinted models. ACS Appl Mater Interfaces, 14: 35344–35356. https://doi.org/10.1021/acsami.2c06658
34. Zennifer A, Manivannan S, Sethuraman S, et al., 2022, 3D bioprinting and photocrosslinking: Emerging strategies and future perspectives. Biomater Adv, 134: 112576. https://doi.org/10.1016/j.msec.2021.112576
35. Matai I, Kaur G, Seyedsalehi A, et al., 2020, Progress in 3D bioprinting technology for tissue/organ regenerative engineering. Biomaterials, 226: 119536. https://doi.org/10.1016/j.biomaterials.2019.119536
36. Li Q, Zhang B, Xue Q, et al., 2021, A systematic thermal analysis for accurately predicting the extrusion printability of alginate-gelatin-based hydrogel bioinks. Int J Bioprint, 7: 394. https://doi.org/10.18063/ijb.v7i3.394
37. Huang Y, Li X, Lu Z, et al., 2020, Nanofiber-reinforced bulk hydrogel: Preparation and structural, mechanical, and biological properties. J Mater Chem B, 8: 9794–9803. https://doi.org/10.1039/d0tb01948h
38. Lai WF, 2021, Development of hydrogels with self-healing properties for delivery of bioactive agents. Mol Pharm, 18: 1833–1841. https://doi.org/10.1021/acs.molpharmaceut.0c00874
39. Naegeli KM, Kural MH, Li Y, et al., 2022, Bioengineering human tissues and the future of vascular replacement. Circ Res, 131(1):109–126. https://doi.org/10.1161/CIRCRESAHA.121.319984
40. Yao ZC, Yang YH, Kong J, et al., 2022, Biostimulatory micro-fragmented nanofiber-hydrogel composite improves mesenchymal stem cell delivery and soft tissue remodeling. Small, 18: e2202309. https://doi.org/10.1002/smll.202202309
41. Xu M, Su T, Jin X, et al., 2022, Inflammation-mediated matrix remodeling of extracellular matrix-mimicking biomaterials in tissue engineering and regenerative medicine. Acta Biomater, 151: 106–117. https://doi.org/10.1016/j.actbio.2022.08.015
42. Pitacco P, Sadowska JM, O’Brien FJ, et al., 2022, 3D bioprinting of cartilaginous templates for large bone defect healing. Acta Biomater, S1742-7061(22)00439-1. https://doi.org/10.1016/j.actbio.2022.07.037
43. Park JH, Jo SB, Lee JH, et al., 2022, Materials and extracellular matrix rigidity highlighted in tissue damages and diseases: Implication for biomaterials design and therapeutic targets. Bioact Mater, 20:381–403. https://doi.org/10.1016/j.bioactmat.2022.06.003
44. Guimarães CF, Marques AP, Reis RL, 2022, Pushing the natural frontier: Progress on the integration of biomaterial cues toward combinatorial biofabrication and tissue engineering. Adv Mater, 34: e2105645. https://doi.org/10.1002/adma.202105645