Relationship between shear-thinning rheological properties of bioinks and bioprinting parameters
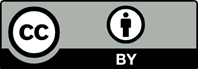
Three-dimensional bioprinting is a technology in constant development, mainly due to its extraordinary potential to revolutionize regenerative medicine. It allows fabrication through the additive deposition of biochemical products, biological materials, and living cells for the generation of structures in bioengineering. There are various techniques and biomaterials or bioinks that are suitable for bioprinting. Their rheological properties are directly related to the quality of these processes. In this study, alginate-based hydrogels were prepared using CaCl2 as ionic crosslinking agent. Their rheological behavior was studied, and simulations of the bioprinting processes under predetermined conditions were carried out, looking for possible relationships between the rheological parameters and the variables used in the bioprinting processes. A clear linear relationship was found between the extrusion pressure and the flow consistency index rheological parameter, k, and between the extrusion time and the flow behavior index rheological parameter, n. This would allow simplification of the repetitive processes currently applied to optimize the extrusion pressure and dispensing head displacement speed, thereby helping to reduce the time and material used as well as to optimize the required bioprinting results.
1. Fontrodona J, Raül F, Díaz B, 2014, Estado Actual y Perspectivas De La Impresión En 3D. Available from: www. cege.es [Last accessed 2021 May 10]
2. Li J, Chen M, Fan X., et al., 2016, Recent advances in bioprinting techniques: Approaches, applications and future prospects. J Transl Med, 14: 271. https://doi.org/10.1186/s12967-016-1028-0
3. Tasnim N, Kumar SA, Joddar B, et al., 2018, 3D bioprinting stem cell derived tissues. Cell Mol Bioeng, 11: 219–240. https://doi.org/10.1007/s12195-018-0530-2
4. Hoch E, Tovar GE, Borchers K, 2014, Bioprinting of artificial blood vessels: Current approaches towards a demanding goal. Eur J Cardiothorac Surg, 46: 767–778.https://doi.org/10.1093/ejcts/ezu242
5. Vijayavenkataraman S, Lu WF, Fuh JY, 2016, 3D bioprinting of skin: A state-of-the-art review on modelling, materials, and processes. Biofabrication, 8: 032001. https://doi.org/10.1088/1758-5090/8/3/032001
6. Cubo N, Garcia M, Del Cañizo JF, et al., 2016, 3D bioprinting of functional human skin: Production and in vivo analysis. Biofabrication, 9: 015006. https://doi.org/10.1088/1758-5090/9/1/015006
7. Izadifar M, Chapman D, Babyn P, et al., 2018, UV-assisted 3D bioprinting of nanoreinforced hybrid cardiac patch for myocardial tissue engineering. Tissue Eng Part C Methods, 24: 74–88. https://doi.org/10.1089/ten.tec.2017.0346
8. Xiongfa J, Hao Z, Liming Z, et al., 2018, Recent advances in 3D bioprinting for the regeneration of functional cartilage. Regen Med, 13: 73–87. https://doi.org/10.2217/rme-2017-0106
9. Wang X, Ao Q, Tian X, et al., 2016, 3D bioprinting technologies for hard tissue and organ engineering. Materials (Basel), 9: 802. https://doi.org/10.3390/ma9100802
10. Ashammakhi N, Hasan A, Kaarela O, et al., 2019, Advancing frontiers in bone bioprinting. Adv Healthc Mater, 8: 1801048. https://doi.org/10.1002/adhm.201801048
11. Derakhshanfar S, Mbeleck R, Xu K, et al., 2018, 3D bioprinting for biomedical devices and tissue engineering: A review of recent trends and advances. Bioact Mater, 3: 144–156. https://doi.org/10.1016/j.bioactmat.2017.11.008
12. Pedroza-González SC, Rodriguez-Salvador M, Pérez-Benítez BE, et al., 2021, Bioinks for 3D bioprinting: A scientometric analysis of two decades of progress. Int J Bioprint, 7: 333. https://doi.org/10.18063/ijb.v7i2.337
13. Mobaraki M, Ghaffari M, Yazdanpanah A, et al., 2020, Bioinks and bioprinting: A focused review. Bioprinting, 18: e00080. https://doi.org/10.1016/j.bprint.2020.e00080
14. Annabi N, Tamayol A, Uquillas JA, et al., 2014, 25th anniversary article: Rational design and applications of hydrogels in regenerative medicine. Adv Mater, 26: 85–123. https://doi.org/10.1002/adma.201303233
15. Fisher OZ, Khademhosseini A, Langer R, et al., 2010, Bioinspired materials for controlling stem cell fate. Acc Chem Res, 43: 419–428. https://doi.org/10.1021/ar900226q
16. Slaughter BV, Khurshid SS, Fisher OZ, et al., 2009, Hydrogels in regenerative medicine. Adv Mater, 21: 3307–3329. https://doi.org/10.1002/adma.200802106
17. Mørch YA, Donati I, Strand BL, et al., 2006, Effect of Ca2+, Ba2+, and Sr2+ on alginate microbeads. Biomacromolecules, 7: 1471–1480. https://doi.org/10.1021/bm060010d
18. Freeman FE, Kelly DJ, 2017, Tuning alginate bioink stiffness and composition for controlled growth factor delivery and to spatially direct MSC Fate within bioprinted tissues. Sci Rep, 7: 17042. https://doi.org/10.1038/s41598-017-17286-1
19. Kim MH, Lee YW, Jung WK, et al., 2019, Nam, Enhanced rheological behaviors of alginate hydrogels with carrageenan for extrusion-based bioprinting. J Mech Behav Biomed Mater, 98: 187–194. https://doi.org/10.1016/j.jmbbm.2019.06.014
20. Gonzalez-Fernandez T, Tenorio AJ, Campbell KT, et al., 2021, Alginate-based bioinks for 3d bioprinting and fabrication of anatomically accurate bone grafts. Tissue Eng Part A, 27: 1168–1181. https://doi.org/10.1089/ten.tea.2020.0305
21. Li H, Tan YJ, Kiran R, et al., 2021, Submerged and non-submerged 3D bioprinting approaches for the fabrication of complex structures with the hydrogel pair GelMA and alginate/methylcellulose. Addit Manufac, 37: 101640. https://doi.org/10.1016/j.addma.2020.101640
22. Li H, Tan YJ, Leong KF, et al., 2017, 3D Bioprinting of highly thixotropic alginate/methylcellulose hydrogel with strong interface bonding. ACS Appl Mater Interfaces, 9: 20086–20097. https://doi.org/10.1021/acsami.7b04216
23. Aldana AA, Valente F, Dilley R, et al., 2021, Development of 3D bioprinted GelMA-alginate hydrogels with tunable mechanical properties. Bioprinting, 21: e00105. https://doi.org/10.1016/j.bprint.2020.e00105
24. Antich C, de Vicente J, Jiménez G, et al., 2020, Bio-inspired hydrogel composed of hyaluronic acid and alginate as a potential bioink for 3D bioprinting of articular cartilage engineering constructs. Acta Biomater, 106: 114–123. https://doi.org/10.1016/j.actbio.2020.01.046
25. Adhikari J, Perwez MS, Das A, et al., 2021, Development of hydroxyapatite reinforced alginate-chitosan based printable biomaterial-ink. Nano Struct Nano Objects. 25: 100630. https://doi.org/10.1016/j.nanoso.2020.100630
26. Mousavi SM, Rafe A, Yeganehzad S, 2020, Structure-rheology relationships of composite gels: Alginate and Basil seed gum/guar gum. Carbohydr Polym, 232: 115809. https://doi.org/10.1016/j.carbpol.2019.115809
27. Karimi H, Javaherdeh K, 2021, Numerical analysis of mix convection of sodium alginate non-Newtonian fluid with Al2O3 nanoparticle in a channel with block. J Appl Comput Sci Mech, 32: 93–110. https://doi.org/10.22067/JACSM.2021.40042
28. Batalha LS, Gontijo MT, de Carvalho AV, et al., 2021, Encapsulation in alginate-polymers improves stability and allows controlled release of the UFV-AREG1 bacteriophage. Food Res Int, 139: 109947. https://doi.org/10.1016/j.foodres.2020.109947
29. Sugiono S, Masruri M, Estiasih T, et al., 2019, Structural and rheological characteristics of alginate from Sargassum cristaefolium extracted by Twin screw extruder. J Aquat Food Prod Technol, 28: 944–959. https://doi.org/10.1080/10498850.2019.1665603
30. Sugiono S, Masruri M, Estiasih T, et al, 2019, Optimization of extrusion-assisted extraction parameters and characterization of alginate from brown algae (Sargassum cristaefolium). J Food Sci Technol, 56: 3687–3696. https://doi.org/10.1007/s13197-019-03829-z
31. Li V, Liu S, Li L, 2016, Rheological study on 3D printability of alginate hydrogel and effect of graphene oxide. Int J Bioprint, 2: 54–66. https://doi.org/10.18063/IJB.2016.02.007
32. Yürüsoy M, Pakdemirli M, 1999, Group classification of a non-Newtonian fluid model using classical approach and equivalence transformations. Int J Non Linear Mech, 34: 341–346. https://doi.org/10.1016/S0020-7462(98)00037-7