3D printable conductive composite inks for the fabrication of biocompatible electrodes in tissue engineering application
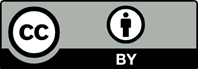
Native tissues are affected by the microenvironment surrounding the tissue, including electrical activities. External electrical stimulation, which is used in replicating electrical activities and regulating cell behavior, is mainly applied in neural and cardiac tissues due to their electrophysiological properties. The in vitro cell culture platform with electrodes provides precise control of the stimulation property and eases the observation of the effects on the cells. The frequently used electrodes are metal or carbon rods, but their risk of damaging tissue and their mechanical properties that are largely different from those of native tissues hinder further applications. Biocompatible polymer reinforced with conductive fillers emerges as a potential solution to fabricate the complex structure of the platform and electrode. Conductive polymer can be used as an ink in the extrusion-based printing method, thus enabling the fabrication of volumetric structures. The filler simultaneously alters the electrical and rheological properties of the ink; therefore, the amount of additional compound should be precisely determined regarding printability and conductivity. This review provides an overview on the rheology and conductivity change relative to the concentration of conductive fillers and the applications of printed electrodes. Next, we discuss the future potential use of a cell culture platform with electrodes from in vitro and in vivo perspectives.
1. Murphy SV, Atala A, 2014, 3D bioprinting of tissues and organs. Nat Biotechnol, 32: 773–785. https://doi.org/10.1038/nbt.2958
2. da Silva LP, Kundu SC, Reis RL, et al., 2020, Electric phenomenon: A disregarded tool in tissue engineering and regenerative medicine. Trends Biotechnol, 38: 24–49. https://doi.org/10.1016/j.tibtech.2019.07.002
3. Chen C, Bai X, Ding Y, et al., 2019, Electrical stimulation as a novel tool for regulating cell behavior in tissue engineering. Biomater Res, 23: 25. https://doi.org/10.1186/s40824-019-0176-8
4. Yuan X, Arkonac DE, Chao PHG, et al., 2014, Electrical stimulation enhances cell migration and integrative repair in the meniscus. Sci Rep, 4: 3674. https://doi.org/10.1038/srep03674
5. Xia Y, Buja LM, Richard CS, et al., 1997, Electrical stimulation of neonatal cardiomyocytes results in the sequential activation of nuclear genes governing mitochondrial proliferation and differentiation. Proc Natl Acad Sci, 94: 11399–11404. https://doi.org/10.1073/pnas.94.21.11399
6. Love MR, Palee S, Chattipakorn SC, et al., 2018, Effects of electrical stimulation on cell proliferation and apoptosis. J Cell Physiol, 233: 1860–1876. https://doi.org/10.1002/jcp.25975
7. Park D, Park J, Lee J, et al., 2021, Fabrication and characterization of graphene oxide-coated plate for efficient culture of stem cells. Tissue Eng Regen Med, 18: 775–785. https://doi.org/10.1007/s13770-021-00370-z
8. Hernández D, Millard R, Sivakumaran P, et al., 2016, Electrical stimulation promotes cardiac differentiation of human induced pluripotent stem cells. Stem Cells Int, 2016: 1718041. https://doi.org/10.1155/2016/1718041
9. Hirt MN, Boeddinghaus J, Mitchell A, et al., 2014, Functional improvement and maturation of rat and human engineered heart tissue by chronic electrical stimulation. J Mol Cell Cardiol, 74: 151–161. https://doi.org/10.1016/j.yjmcc.2014.05.009
10. LaBarge W, Mattappally S, Kannappan R, et al., 2019, Maturation of three-dimensional, hipsc-derived cardiomyocyte spheroids utilizing cyclic, uniaxial stretch and electrical stimulation. PLoS One, 14: e0219442. https://doi.org/10.1371/journal.pone.0219442
11. Sadeghian RB, Ebrahimi M, Salehi S, 2018, Electrical stimulation of micro engineered skeletal muscle tissue: Effect of stimulus parameters on myotube contractility and maturation. J Tissue Eng Regen Med, 12: 912–922. https://doi.org/10.1002/term.2502
12. Tandon N, Cannizzaro C, Chao PH, et al., 2009, Electrical stimulation systems for cardiac tissue engineering. Nat Protoc, 4: 155–173. https://doi.org/10.1038/nprot.2008.183
13. Harris AR, 2020, Current perspectives on the safe electrical stimulation of peripheral nerves with platinum electrodes. Bioelectron Med, 3: 37–49. https://doi.org/10.2217/bem-2020-0007
14. Brummer SB, Turner MJ, 1977, Electrochemical considerations for safe electrical stimulation of the nervous system with platinum electrodes. IEEE Trans Biomed Eng, 24: 59–63. https://doi.org/10.1109/TBME.1977.326218
15. Khaw JS, Xue R, Cassidy NJ, et al., 2022, Electrical stimulation of titanium to promote stem cell orientation, elongation and osteogenesis. Acta Biomater, 139: 204–217. https://doi.org/10.1016/j.actbio.2021.08.010
16. Shepherd RK, Carter PM, Dalrymple AN, et al., 2021, Platinum dissolution and tissue response following longterm electrical stimulation at high charge densities. J Neural Eng, 18: 036021. https://doi.org/10.1088/1741-2552/abe5ba
17. Harnack D, Winter C, Meissner W, et al., 2004, The effects of electrode material, charge density and stimulation duration on the safety of high-frequency stimulation of the subthalamic nucleus in rats. J Neurosci Methods, 138: 207–216. https://doi.org/10.1016/j.jneumeth.2004.04.019
18. Ngo TD, Kashani A, Imbalzano G, et al., 2018, Additive manufacturing (3D Printing): A review of materials, methods, applications and challenges. Compos Part B Eng, 143: 172–196. https://doi.org/10.1016/j.compositesb.2018.02.012
19. Ravanbakhsh H, Bao G, Luo Z, et al., 2021, Composite inks for extrusion printing of biological and biomedicalconstructs. ACS Biomater Sci Eng, 7: 4009–4026. https://doi.org/10.1021/acsbiomaterials.0c01158
20. Guimarães CF, Gasperini L, Marques AP, et al., 2020, The Stiffness of living tissues and its implications for tissue engineering. Nat Rev Mater, 5: 351–370. https://doi.org/10.1038/s41578-019-0169-1
21. Sun X, Sun H, Li H, et al., 2013, Developing polymer composite materials: Carbon nanotubes or graphene? Adv Mater, 25: 5153–5176. https://doi.org/10.1002/adma.201301926
22. Schiavone G, Kang X, Fallegger F, et al., 2020, Guidelines to study and develop soft electrode systems for neural stimulation. Neuron, 108: 238–258. https://doi.org/10.1016/j.neuron.2020.10.010
23. Chen FM, Liu X, 2016, Advancing biomaterials of human origin for tissue engineering. Prog Polym Sci, 53: 86–168. https://doi.org/10.1016/j.progpolymsci.2015.02.004
24. Christopherson GT, de Vasconcellos JF, Dunn JC, et al., 2021, Three-dimensional modeling of the structural microenvironment in post-traumatic war wounds. Tissue Eng Regen Med, 18: 963–973. https://doi.org/10.1007/s13770-021-00355-y
25. Skylar-Scott MA, Mueller J, Visser CW, et al., 2019, Voxelated soft matter via multimaterial multinozzle 3D printing. Nature, 575: 330–335. https://doi.org/10.1038/s41586-019-1736-8
26. Gillispie G, Prim P, Copus J, et al., 2020, Assessment methodologies for extrusion-based bioink printability. Biofabrication, 12: 022003.https://doi.org/10.1088/1758-5090/ab6f0d. Franco JM, Partal P, 2010, The newtonian fluid. Rheology, 1: 74–95.
28. Viswanath DS, Ghosh TK, Prasad DH, et al., 2007, Viscosity of liquids: theory, estimation, experiment, and data. Berlin, germany: Springer science and business media.
29. Cooke ME, Rosenzweig DH, 2021, The rheology of direct and suspended extrusion bioprinting. APL Bioeng, 5: 011502. https://doi.org/10.1063/5.0031475
30. Ferry JD, 1980, Viscoelastic properties of polymers. Hoboken, new jersey: John Wiley and Sons.
31. Mewis J, Wagner NJ, 2009, Thixotropy. Adv Colloid Interface Sci, 147–148: 214–227. https://doi.org/10.1016/j.cis.2008.09.005
32. Huang YY, Terentjev EM, 2012, Dispersion of carbon nanotubes: Mixing, sonication, stabilization, and composite properties. Polymers, 4: 275–295.
33. Lewis JA, 2000, Colloidal processing of ceramics. J Am Ceram Soc, 83:2341–59. https://doi.org/10.1111/j.1151-2916.2000.tb01560.x
34. Ma PC, Siddiqui NA, Marom G, et al., 2010, Dispersion and functionalization of carbon nanotubes for polymer-based nanocomposites: a review. Compos Part A: Appl Sci Manuf, 41: 1345–1367. https://doi.org/10.1016/j.compositesa.2010.07.003
35. Li MC, Wu Q, Moon RJ, et al., 2021, Rheological aspects of cellulose nanomaterials: Governing factors and emerging applications. Adv Mater, 33: 2006052. https://doi.org/10.1002/adma.202006052
36. Genovese DB, 2012, Shear rheology of hard-sphere, dispersed, and aggregated suspensions, and filler-matrix composites. Adv Colloid Interface Sci, 171–2: 1–16. https://doi.org/10.1016/j.cis.2011.12.005
37. Rueda MM, Auscher MC, Fulchiron R, et al., 2017, Rheology and applications of highly filled polymers: A review of current understanding. Prog Polym Sci, 66: 22–53. https://doi.org/10.1016/j.progpolymsci.2016.12.007
38. O’ Mahony C, Haq EU, Silien C, et al., 2019, Rheological issues in carbon-based inks for additive manufacturing. Micromachines (Basel), 10: 99. https://doi.org/10.3390/mi10020099
39. Fonseca FC, Muccillo R, de Florio DZ, et al., 2007, Mixed ionic-electronic conductivity in yttria-stabilized zirconia/carbon nanotube composites. Appl Phys Lett, 91: 243107. https://doi.org/10.1063/1.2821373
40. Mohd Radzuan NA, Sulong AB, Sahari J, 2017, A review of electrical conductivity models for conductive polymer composite. Int J Hydrogen Energy, 42: 9262–9273. https://doi.org/10.1016/j.ijhydene.2016.03.045
41. Li C, Thostenson ET, Chou TW, 2007, Dominant role of tunneling resistance in the electrical conductivity of carbon nanotube–based
composites. Appl Phys Lett, 91: 223114. https://doi.org/10.1063/1.2819690
42. Berhan L, Sastry AM, 2007, Modeling Percolation in High-Aspect-Ratio Fiber Systems. I. Soft-Core Versus Hard-Core Models. Phys Rev E, 75:041120. https://doi.org/10.1103/PhysRevE.75.041120
43. Bauhofer W, Kovacs JZ, 2009, A review and analysis of electrical percolation in carbon nanotube polymer composites. Compos Sci Technol, 69: 1486–1498. https://doi.org/10.1016/j.compscitech.2008.06.018
44. Leblanc JL, 2002, Rubber-filler interactions and rheological properties in filled compounds. Prog Polym Sci, 27: 627–687. https://doi.org/10.1016/S0079-6700(01)00040-5
45. Ravanbakhsh H, Bao G, Latifi N, et al., 2019, Carbon nanotube composite hydrogels for vocal fold tissue engineering: biocompatibility, rheology, and porosity. Mater Sci Eng C, 103: 109861. https://doi.org/10.1016/j.msec.2019.109861
46. Islam RR, Md. Hasan A, Md. Abu J, et al., 2019, Carbon nanotubes agglomeration in reinforced composites: A review. AIMS Mater Sci, 6: 756–780. https://doi.org/10.3934/matersci.2019.5.756
47. Mora A, Verma P, Kumar S, 2020, Electrical conductivity of cnt/polymer composites: 3D printing, measurements and modeling. Compos Part B Eng, 183: 107600.
https://doi.org/10.1016/j.compositesb.2019.107600
48. Gong S, Zhu ZH, Li J, et al., 2014, Modeling and characterization of carbon nanotube agglomeration effect on electrical conductivity of carbon nanotube polymer composites. J Appl Phys, 116: 194306. https://doi.org/10.1063/1.4902175
49. Liu CX, Choi JW, 2012, Improved dispersion of carbon nanotubes in polymers at high concentrations. Nanomaterials, 2: 329–347.
50. Zhu K, Shin SR, van Kempen T, et al., 2017, Gold nanocomposite bioink for printing 3D cardiac constructs. Adv Funct Mater, 27: 1605352. https://doi.org/10.1002/adfm.201605352
51. Sahoo NG, Rana S, Cho JW, et al., 2010, Polymer nanocomposites based on functionalized carbon nanotubes. Prog Polym Sci, 35: 837–867. https://doi.org/10.1016/j.progpolymsci.2010.03.002
52. Pidcock GC, in het Panhuis M, 2012, Extrusion printing of flexible electrically conducting carbon nanotube networks. Adv Funct Mater, 22: 4790–4800. https://doi.org/10.1002/adfm.201200724
53. Punetha VD, Rana S, Yoo HJ, et al., 2017, Functionalization of carbon nanomaterials for advanced polymer nanocomposites: a comparison study between cnt and graphene. Prog Polym Sci, 67: 1–47. https://doi.org/10.1016/j.progpolymsci.2016.12.010
54. Ravanbakhsh H, Bao G, Mongeau L, 2020, Carbon nanotubes promote cell migration in hydrogels. Sci Rep, 10: 2543. https://doi.org/10.1038/s41598-020-59463-9
55. Jung I, Jo YH, Kim I, et al., 2012, A simple process for synthesis of ag nanoparticlesand sintering of conductive ink for use in printed electronics. J Electron Mater, 41: 115–21.
https://doi.org/10.1007/s11664-011-1761-3
56. Li Y, Pavanram P, Zhou J, et al., 2020, Additively manufactured biodegradable porous zinc. Acta Biomater, 101: 609–23. https://doi.org/10.1016/j.actbio.2019.10.034
57. Ahn BY, Duoss EB, Motala MJ, et al., 2009, Omnidirectional printing of flexible, stretchable, and spanning silver microelectrodes. Science, 323: 1590–1593. https://doi.org/10.1126/science.1168375
58. Britton J, Krukiewicz K, Chandran M, et al., 2021, A flexible strain-responsive sensor fabricated from a biocompatible electronic ink via an additive-manufacturing process. Mater Des, 206: 109700. https://doi.org/10.1016/j.matdes.2021.109700
59. Farizhandi AA, Khalajabadi SZ, Krishnadoss V, et al., 2020, Synthesized biocompatible and conductive ink for 3D printing of flexible electronics. J Mech Behav Biomed Mater, 110: 103960. https://doi.org/10.1016/j.jmbbm.2020.103960
60. Doh J, Lee J, 2016, Prediction of the mechanical behavior of double walled-cnts using a molecular mechanics-based finite element method: effects of chirality. Comput Struct, 169: 91–100. https://doi.org/10.1016/j.compstruc.2016.03.006
61. Doh J, Park SI, Yang Q, et al., 2019, The effect of carbon nanotube chirality on the electrical conductivity of polymer nanocomposites considering tunneling resistance. Nanotechnology, 30: 465701. https://doi.org/10.1088/1361-6528/ab3b79
62. Giavasis I, Harvey LM, McNeil B, 2000, Gellan gum. Crit Rev Biotechnol, 20: 177–211. https://doi.org/10.1080/07388550008984169
63. Pedrotty DM, Kuzmenko V, Karabulut E, et al., 2019, Three-dimensional printed biopatches with conductive ink facilitate cardiac conduction when applied to disrupted myocardium. Circ: Arrhythm Electrophysiol, 12: e006920. https://doi.org/10.1161/CIRCEP.118.006920
64. Jakus AE, Secor EB, Rutz AL, et al., 2015, Three-dimensional printing of high-content graphene scaffolds for electronic and biomedical applications. ACS Nano, 9: 4636–4648. https://doi.org/10.1021/acsnano.5b01179
65. García-Tuñón E, Feilden E, Zheng H, et al., 2017, Graphene oxide: an all-in-one processing additive for 3D printing. ACS Appl Mater Interfaces, 9: 32977–32989. https://doi.org/10.1021/acsami.7b07717
66. Shi G, Lowe SE, Teo AJ, et al., 2019, A versatile PDMS submicrobead/graphene oxide nanocomposite ink for the direct ink writing of wearable micron-scale tactile sensors. Appl Mater Today, 16: 482–492. https://doi.org/10.1016/j.apmt.2019.06.016
67. Boularaoui S, Shanti A, Lanotte M, et al., 2021, Nanocomposite conductive bioinks based on low-concentration GelMA and mxene nanosheets/gold nanoparticles providing enhanced printability of functional skeletal muscle tissues. ACS Biomater Sci Eng, 7: 5810–5822. https://doi.org/10.1021/acsbiomaterials.1c01193
68. Shin SR, Farzad R, Tamayol A, et al., 2016, A bioactive carbon nanotube-based ink for printing 2D and 3D flexible electronics. Adv Mater, 28: 3280–3289. https://doi.org/10.1002/adma.201506420
69. Bordoni M, Karabulut E, Kuzmenko V, et al., 2020, 3D printed conductive nanocellulose scaffolds for the differentiation of human neuroblastoma cells. Cells, 9: 682. https://doi.org/10.3390/cells9030682
70. Park J, Jeon N, Lee S, et al., 2022, Conductive hydrogel constructs with three-dimensionally connected graphene networks for biomedical applications. Chem Eng J, 446: 137344. https://doi.org/10.1016/j.cej.2022.137344
71. Asulin M, Michael I, Shapira A, et al., 2021, One-step 3D printing of heart patches with built-in electronics for performance regulation. Adv Sci, 8: 2004205. https://doi.org/10.1002/advs.202004205
72. Lind JU, Busbee TA, Valentine AD, et al., 2017, Instrumented cardiac microphysiological devices via multimaterial three dimensional printing. Nat Mater, 16: 303–308. https://doi.org/10.1038/nmat4782
73. Orangi J, Hamade F, Davis VA, et al., 2020, 3D printing of additive-free 2D Ti3C2Tx (MXene) ink for fabrication of micro-supercapacitors with ultra-high energy densities. ACS Nano, 14: 640–650. https://doi.org/10.1021/acsnano.9b07325
74. Naguib M, Mochalin VN, Barsoum MW, et al., 2014, 25th anniversary article: MXenes: A new family of two dimensional materials. Adv Mater, 26: 992–1005. https://doi.org/10.1002/adma.201304138
75. Nasrallah GK, Al-Asmakh M, Rasool K, et al., 2018, Ecotoxicological assessment of Ti3C2Tx (MXene) using a zebrafish embryo model. Environ Sci Nano, 5: 1002–1011. https://doi.org/10.1039/C7EN01239J
76. Muth JT, Vogt DM, Truby RL, et al., 2014, Embedded 3D printing of strain sensors within highly stretchable elastomers. Adv Mater, 26: 6307–6312. https://doi.org/10.1002/adma.201400334
77. Merrill DR, 2010, The electrochemistry of charge injection at the electrode/tissue interface. In: Zhou d, greenbaum e, editors. Implantable neural prostheses 2: Techniques and engineering approaches. New York: Springer. p85–p138.
78. Merrill DR, Bikson M, Jefferys JG, 2005, Electrical stimulation of excitable tissue: Design of efficacious and safe protocols. J Neurosci Methods, 141: 171–198. https://doi.org/10.1016/j.jneumeth.2004.10.020
79. Nunes SS, Miklas JW, Liu J, et al., 2013, Biowire: A platform for maturation of human pluripotent stem cell-derived cardiomyocytes. Nat Methods, 10: 781–787. https://doi.org/10.1038/nmeth.2524
80. Shakeel M, Khan WA, Rahman K, 2017, Fabrication of cost effective and high sensitivity resistive strain gauge using diw technique. Sens Actuators A Phys, 258: 123–130. https://doi.org/10.1016/j.sna.2017.03.003
81. Leppik L, Oliveira KM, Bhavsar MB, et al., 2020, Electrical stimulation in bone tissue engineering treatments. Eur J Trauma Emerg Surg, 46: 231–244. https://doi.org/10.1007/s00068-020-01324-1
82. Talikowska M, Fu X, Lisak G, 2019, Application of conducting polymers to wound care and skin tissue engineering: A review. Biosens Bioelectron, 135: 50–63. https://doi.org/10.1016/j.bios.2019.04.001
83. Liebman C, Vu TM, Phillips A, et al., 2021, Altered β-cell calcium dynamics via electric field exposure. Ann Biomed Eng, 49: 106–114. https://doi.org/10.1007/s10439-020-02517-w
84. Yu J, Zhang Y, Yan J, et al., 2018, Advances in bio responsive closed-loop drug delivery systems. Int J Pharm, 544: 350–357. https://doi.org/10.1016/j.ijpharm.2017.11.064
85. Tetsuka H, Pirrami L, Wang T, et al., 2022, Wirelessly powered 3D printed hierarchical biohybrid robots with multiscale mechanical properties. Adv Funct Mater, 32: 2202674. https://doi.org/10.1002/adfm.202202674
86. Mirvakili SM, Langer R, 2021, Wireless on-demand drug delivery. Nat Electron, 4:464–77. https://doi.org/10.1038/s41928-021-00614-9
87. Sun P, Zhang J, Zhu X, et al., Directly printed interconnection wires between layers for 3D integrated stretchable electronics. Adv Mater Technol, 7: 2200302. https://doi.org/10.1002/admt.202200302