A 3D bioprinted tumor model fabricated with gelatin/sodium alginate/decellularized extracellular matrix bioink
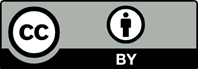
Tissue-engineered scaffolds are more commonly used to construct three-dimensional (3D) tumor models for in vitro studies when compared to the conventional two-dimensional (2D) cell culture because the microenvironments provided by the 3D tumor models closely resemble the in vivo system and could achieve higher success rate when the scaffolds are translated for use in pre-clinical animal model. Physical properties, heterogeneity, and cell behaviors of the model could be regulated to simulate different tumors by changing the components and concentrations of materials. In this study, a novel 3D breast tumor model was fabricated by bioprinting using a bioink that consists of porcine liver-derived decellularized extracellular matrix (dECM) with different concentrations of gelatin and sodium alginate. Primary cells were removed while extracellular matrix components of porcine liver were preserved. The rheological properties of biomimetic bioinks and the physical properties of hybrid scaffolds were investigated, and we found that the addition of gelatin increased hydrophilia and viscoelasticity, while the addition of alginate increased mechanical properties and porosity. The swelling ratio, compression modulus, and porosity could reach 835.43 ± 130.61%, 9.64 ± 0.41 kPa, and 76.62 ± 4.43%, respectively. L929 cells and the mouse breast tumor cells 4T1 were subsequently inoculated to evaluate biocompatibility of the scaffolds and to form the 3D models. The results showed that all scaffolds exhibited good biocompatibility, and the average diameter of tumor spheres could reach 148.52 ± 8.02 μm on 7 d. These findings suggest that the 3D breast tumor model could serve as an effective platform for anticancer drug screening and cancer research in vitro.
Ferlay J, Soerjomataram I, Dikshit R, et al., 2015, Cancer incidence and mortality worldwide: Sources, methods and major patterns in GLOBOCAN 2012. Int J Cancer, 136(5): E359–86. https://doi.org/10.1002/ijc.29210
Ferlay J, Colombet M, Soerjomataram I, et al., 2021, Cancer statistics for the year 2020: An overview. Int J Cancer, 149(8): 778–789. https://doi.org/10.1002/ijc.33588
Xin X, Yang H, Zhang F, et al., 2019, 3D cell coculture tumor model: A promising approach for future cancer drug discovery. Process Biochem, 78: 148–160. https://doi.org/10.1016/j.procbio.2018.12.028
Fong EL, Harrington DA, Farach-Carson MC, et al., 2016, Heralding a new paradigm in 3D tumor modeling. Biomaterials, 108: 197–213. https://doi.org/10.1016/j.biomaterials.2016.08.052
Zhang C, Yang Z, Dong DL, et al., 2020, 3D culture technologies of cancer stem cells: promising ex vivo tumor models. J Tissue Eng, 11: 1–17. https://doi.org/10.1177/2041731420933407
Shapira A, Dvir T, 2021, 3D tissue and organ printing-hope and reality. Adv Sci, 8(10): 2003751. https://doi.org/10.1002/advs.202003751
Ng WL, Chua CK, Shen Y-F, 2019, Print me an organ! Why we are not there yet. Prog Polym Sci, 97: 101145. https://doi.org/10.1016/j.progpolymsci.2019.101145
Ma L, Li Y, Wu Y, et al., 2020, The construction of in vitro tumor models based on 3D bioprinting. Bio-Des Manuf, 3(3): 227–236. https://doi.org/10.1007/s42242-020-00068-6
Mao S, Pang Y, Liu T, et al., 2020, Bioprinting of in vitro tumor models for personalized cancer treatment: a review. Biofabrication, 12(4): 042001. https://doi.org/10.1088/1758-5090/ab97c0
Xie F, Sun L, Pang Y, et al., 2021, Three-dimensional bio-printing of primary human hepatocellular carcinoma for personalized medicine. Biomaterials, 265: 120416. https://doi.org/10.1016/j.biomaterials.2020.120416
Bahcecioglu G, Basara G, Ellis BW, et al., 2020, Breast cancer models: Engineering the tumor microenvironment. Acta Biomater, 106: 1–21. https://doi.org/10.1016/j.actbio.2020.02.006
Jiang T, Munguia-Lopez JG, Flores-Torres S, et al., 2019, Extrusion bioprinting of soft materials: An emerging technique for biological model fabrication. Appl Phys Rev, 6(1): 011310. https://doi.org/10.1063/1.5059393
Li X, Liu B, Pei B, et al., 2020, Inkjet bioprinting of biomaterials. Chem Rev, 120(19): 10793–10833. https://doi.org/10.1021/acs.chemrev.0c00008
Ng WL, Lee JM, Zhou M, et al., 2020, Vat polymerization-based bioprinting-process, materials, applications and regulatory challenges. Biofabrication, 12(2): 022001. https://doi.org/10.1088/1758-5090/ab6034
Oztan YC, Nawafleh N, Zhou Y, et al., 2020, Recent advances on utilization of bioprinting for tumor modeling. Bioprinting, 18: e00079. https://doi.org/10.1016/j.bprint.2020.e00079
Mazza G, Telese A, Al-Akkad W, et al., 2019, Cirrhotic human liver extracellular matrix 3d scaffolds promote smad-dependent tgf-beta1 epithelial mesenchymal transition. Cells, 9(1): 83. https://doi.org/10.3390/cells9010083
Hoshiba T, 2019, Decellularized extracellular matrix for cancer research. Materials (Basel), 12(8): 1311. https://doi.org/10.3390/ma12081311
Kabirian F, Mozafari M, 2020, Decellularized ECM-derived bioinks: Prospects for the future. Methods, 171: 108–118. https://doi.org/10.1016/j.ymeth.2019.04.019
Su J, Satchell SC, Wertheim JA, et al., 2019, Poly(ethylene glycol)-crosslinked gelatin hydrogel substrates with conjugated bioactive peptides influence endothelial cell behavior. Biomaterials, 201: 99–112. https://doi.org/10.1016/j.biomaterials.2019.02.001
Estrada MF, Rebelo SP, Davies EJ, et al., 2016, Modelling the tumour microenvironment in long-term microencapsulated 3D co-cultures recapitulates phenotypic features of disease progression. Biomaterials, 78: 50–61. https://doi.org/10.1016/j.biomaterials.2015.11.030
Xu K, Wang Z, Copland JA, et al., 2020, 3D porous chitosan-chondroitin sulfate scaffolds promote epithelial to mesenchymal transition in prostate cancer cells. Biomaterials, 254: 120126. https://doi.org/10.1016/j.biomaterials.2020.120126
Choi S, Friedrichs J, Song YH, et al., 2019, Intrafibrillar, bone-mimetic collagen mineralization regulates breast cancer cell adhesion and migration. Biomaterials, 198: 95–106. https://doi.org/10.1016/j.biomaterials.2018.05.002
Alabi BR, Laranger R, Shay JW, 2019, Decellularized mice colons as models to study the contribution of the extracellular matrix to cell behavior and colon cancer progression. Acta Biomater, 100: 213–222. https://doi.org/10.1016/j.actbio.2019.09.033
Suo A, Xu W, Wang Y, et al., 2019, Dual-degradable and injectable hyaluronic acid hydrogel mimicking extracellular matrix for 3D culture of breast cancer MCF-7 cells. Carbohydr Polym, 211: 336–348. https://doi.org/10.1016/j.carbpol.2019.01.115
Zhang T, Zhang Q, Chen J, et al., 2014, The controllable preparation of porous PLGA microspheres by the oil/ water emulsion method and its application in 3D culture of ovarian cancer cells. Colloids Surf Physicochem Eng Aspects, 452: 115–124. https://doi.org/10.1016/j.colsurfa.2014.03.085
Ariadna G-P, Marc R, Teresa P, et al., 2016, Optimization of poli(ɛ-caprolactone) scaffolds suitable for 3D cancer cell culture. Procedia CIRP, 49: 61–66. https://doi.org/10.1016/j.procir.2015.07.031
Wang C, Li J, Sinha S, et al., 2019, Mimicking brain tumor-vasculature microanatomical architecture via co-culture of brain tumor and endothelial cells in 3D hydrogels. Biomaterials, 202: 35–44. https://doi.org/10.1016/j.biomaterials.2019.02.024
Ferreira LP, Gaspar VM, Mano JF, 2020, Decellularized extracellular matrix for bioengineering physiomimetic 3D in vitro tumor models. Trends Biotechnol, 38(12): 1397– 1414. https://doi.org/10.1016/j.tibtech.2020.04.006
Zhao L, Huang L, Yu S, et al., 2017, Decellularized tongue tissue as an in vitro model for studying tongue cancer and tongue regeneration. Acta Biomater, 58: 122–135. https://doi.org/10.1016/j.actbio.2017.05.062
Narkhede AA, Shevde LA, Rao SS, 2017, Biomimetic strategies to recapitulate organ specific microenvironments for studying breast cancer metastasis. Int J Cancer, 141(6): 1091–1109. https://doi.org/10.1002/ijc.30748
Lang R, Stern MM, Smith L, et al., 2011, Three-dimensional culture of hepatocytes on porcine liver tissue-derived extracellular matrix. Biomaterials, 32(29): 7042–7052. https://doi.org/10.1016/j.biomaterials.2011.06.005
Zhao F, Cheng J, Sun M, et al., 2020, Digestion degree is a key factor to regulate the printability of pure tendon decellularized extracellular matrix bio-ink in extrusion-based 3D cell printing. Biofabrication, 12(4): 045011. https://doi.org/10.1088/1758-5090/aba411
Pati F, Jang J, Ha DH, et al., 2014, Printing three-dimensional tissue analogues with decellularized extracellular matrix bioink. Nat Commun, 5: 3935. https://doi.org/10.1038/ncomms4935
Xu J, Fang H, Zheng S, et al., 2021, A biological functional hybrid scaffold based on decellularized extracellular matrix/ gelatin/chitosan with high biocompatibility and antibacterial activity for skin tissue engineering. Int J Biol Macromol, 187: 840–849. https://doi.org/10.1016/j.ijbiomac.2021.07.162
Kim BS, Kwon YW, Kong JS, et al., 2018, 3D cell printing of in vitro stabilized skin model and in vivo pre-vascularized skin patch using tissue-specific extracellular matrix bioink: A step towards advanced skin tissue engineering. Biomaterials, 168: 38–53. https://doi.org/10.1016/j.biomaterials.2018.03.040
Kim J, Shim IK, Hwang DG, et al., 2019, 3D cell printing of islet-laden pancreatic tissue-derived extracellular matrix bioink constructs for enhancing pancreatic functions. J Mater Chem B, 7(10): 1773–1781. https://doi.org/10.1039/c8tb02787k
Xu J, Fang H, Su Y, et al., 2022, A 3D bioprinted decellularized extracellular matrix/gelatin/quaternized chitosan scaffold assembling with poly(ionic liquid)s for skin tissue engineering. Int J Biol Macromol, 220: 1253–1266. https://doi.org/10.1016/j.ijbiomac.2022.08.149
Zhang Y, Yuan B, Zhang Y, et al., 2020, Biomimetic lignin/ poly(ionic liquids) composite hydrogel dressing with excellent mechanical strength, self-healing properties, and reusability. Chem Eng J, 400: 125984. https://doi.org/10.1016/j.cej.2020.125984
Du J, Hu X, Su Y, et al., 2022, Gelatin/sodium alginate hydrogel-coated decellularized porcine coronary artery to construct bilayer tissue engineered blood vessels. Int J Biol Macromol, 209: 2070–2083. https://doi.org/10.1016/j.ijbiomac.2022.04.188
Coronado RE, Somaraki-Cormier M, Natesan S, et al., 2018, Decellularization and solubilization of porcine liver for use as a substrate for porcine hepatocyte culture. Cell Transplant, 26(12): 1840–1854. https://doi.org/10.1177/0963689717742157
Abaci A, Guvendiren M, 2020, Designing decellularized extracellular matrix-based bioinks for 3D bioprinting. Adv Healthc Mater, 9(24): e2000734. https://doi.org/10.1002/adhm.202000734
Sellaro TL, Ranade A, Faulk DM, et al., 2010, Maintenance of human hepatocyte function in vitro by liver-derived extracellular matrix gels. Tissue Eng Part A, 16(3): 1075– 1082. https://doi.org/10.1089/ten.tea.2008.0587
Saleh T, Ahmed E, Yu L, et al., 2018, Silver nanoparticles improve structural stability and biocompatibility of decellularized porcine liver. Artif Cells Nanomed Biotechnol, 46(sup2): 273–284. https://doi.org/10.1080/21691401.2018.1457037
Wu Q, Bao J, Zhou YJ, et al., 2015, Optimizing perfusion-decellularization methods of porcine livers for clinical-scale whole-organ bioengineering. Biomed Res Int, 2015: 785474. https://doi.org/10.1155/2015/785474
Poornejad N, Nielsen JJ, Morris RJ, et al., 2016, Comparison of four decontamination treatments on porcine renal decellularized extracellular matrix structure, composition, and support of human renal cortical tubular epithelium cells. J Biomater Appl, 30(8): 1154–1167. https://doi.org/10.1177/0885328215615760
Struecker B, Hillebrandt KH, Voitl R, et al., 2015, Porcine liver decellularization under oscillating pressure conditions: A technical refinement to improve the homogeneity of the decellularization process. Tissue Eng Part C Methods, 21(3): 303–313. https://doi.org/10.1089/ten.TEC.2014.0321
Sun D, Liu Y, Wang H, et al., 2018, Novel decellularized liver matrix-alginate hybrid gel beads for the 3D culture of hepatocellular carcinoma cells. Int J Biol Macromol, 109: 1154–1163. https://doi.org/10.1016/j.ijbiomac.2017.11.103
Hu X, Li W, Li L, et al., 2019, A biomimetic cartilage gradient hybrid scaffold for functional tissue engineering of cartilage. Tissue Cell, 58: 84–92. https://doi.org/10.1016/j.tice.2019.05.001
Li W, Hu X, Yang S, et al., 2018, A novel tissue-engineered 3D tumor model for anti-cancer drug discovery. Biofabrication, 11(1): 015004. https://doi.org/10.1088/1758-5090/aae270
Mao S, He J, Zhao Y, et al., 2020, Bioprinting of patient-derived in vitro intrahepatic cholangiocarcinoma tumor model: Establishment, evaluation and anti-cancer drug testing. Biofabrication, 12(4): 045014. https://doi.org/10.1088/1758-5090/aba0c3
Song K, Li L, Yan X, et al., 2017, Characterization of human adipose tissue-derived stem cells in vitro culture and in vivo differentiation in a temperature-sensitive chitosan/beta-glycerophosphate/collagen hybrid hydrogel. Mater Sci Eng C Mater Biol Appl, 70(Pt 1): 231–240. https://doi.org/10.1016/j.msec.2016.08.085
Ijima H, Nakamura S, Bual R, et al., 2018, Physical properties of the extracellular matrix of decellularized porcine liver. Gels, 4(2): 39. https://doi.org/10.3390/gels4020039
Polley C, Mau R, Lieberwirth C, et al., 2017, Bioprinting of three dimensional tumor models: a preliminary study using a low cost 3D printer. Curr Dir Biomed Eng, 3(2): 135–138. https://doi.org/10.1515/cdbme-2017-0028
Lee H, Han W, Kim H, et al., 2017, Development of liver decellularized extracellular matrix bioink for three-dimensional cell printing-based liver tissue engineering. Biomacromolecules, 18(4): 1229–1237. https://doi.org/10.1021/acs.biomac.6b01908
Chaji S, Al-Saleh J, Gomillion CT, 2020, Bioprinted three-dimensional cell-laden hydrogels to evaluate adipocyte-breast cancer cell interactions. Gels, 6(1): 10. https://doi.org/10.3390/gels6010010
Lv K, Zhu J, Zheng S, et al., 2021, Evaluation of inhibitory effects of geniposide on a tumor model of human breast cancer based on 3D printed Cs/Gel hybrid scaffold. Mater Sci Eng C, 119: 111509. https://doi.org/10.1016/j.msec.2020.111509
Jeong W, Kim MK, Kang HW, 2021, Effect of detergent type on the performance of liver decellularized extracellular matrix-based bio-inks. J Tissue Eng, 12: 1–14. https://doi.org/10.1177/2041731421997091
Bordoni M, Karabulut E, Kuzmenko V, et al., 2020, 3D printed conductive nanocellulose scaffolds for the differentiation of human neuroblastoma cells. Cells, 9(3): 682. https://doi.org/10.3390/cells9030682
Zhao C, Li Y, Peng G, et al., 2020, Decellularized liver matrix-modified chitosan fibrous scaffold as a substrate for C3A hepatocyte culture. J Biomater Sci, Polym Ed, 31(8): 1041–1056. https://doi.org/10.1080/09205063.2020.1738690
Lu S, Cuzzucoli F, Jiang J, et al., 2018, Development of a biomimetic liver tumor-on-a-chip model based on decellularized liver matrix for toxicity testing. Lab Chip, 18(22): 3379–3392. https://doi.org/10.1039/c8lc00852c
Wang JZ, Zhu YX, Ma HC, et al., 2016, Developing multi-cellular tumor spheroid model (MCTS) in the chitosan/ collagen/alginate (CCA) fibrous scaffold for anticancer drug screening. Mater Sci Eng C Mater Biol Appl, 62: 215–225. https://doi.org/10.1016/j.msec.2016.01.045
Sachlos E, Reis N, Ainsley C, et al., 2003, Novel collagen scaffolds with predefined internal morphology made by solid freeform fabrication. Biomaterials, 24(8): 1487–1497. https://doi.org/10.1016/S0142-9612(02)00528-8
Xu W, Qian J, Zhang Y, et al., 2016, A double-network poly(Nvarepsilon-acryloyl L-lysine)/hyaluronic acid hydrogel as a mimic of the breast tumor microenvironment. Acta Biomater, 33: 131–141. https://doi.org/10.1016/j.actbio.2016.01.027
Li X, Deng Q, Zhuang T, et al., 2020, 3D bioprinted breast tumor model for structure–activity relationship study. Bio- Des Manuf, 3(4): 361–372. https://doi.org/10.1007/s42242-020-00085-5
Peela N, Sam FS, Christenson W, et al., 2016, A three dimensional micropatterned tumor model for breast cancer cell migration studies. Biomaterials, 81: 72–83. https://doi.org/10.1016/j.biomaterials.2015.11.039
Antunes J, Gaspar VM, Ferreira L, et al., 2019, In-air production of 3D co-culture tumor spheroid hydrogels for expedited drug screening. Acta Biomater, 94: 392–409. https://doi.org/10.1016/j.actbio.2019.06.012
Li L, Qin S, Peng J, et al., 2020, Engineering gelatin-based alginate/carbon nanotubes blend bioink for direct 3D printing of vessel constructs. Int J Biol Macromol, 145: 262–271. https://doi.org/10.1016/j.ijbiomac.2019.12.174
Xu K, Ganapathy K, Andl T, et al., 2019, 3D porous chitosan-alginate scaffold stiffness promotes differential responses in prostate cancer cell lines. Biomaterials, 217: 119311. https://doi.org/10.1016/j.biomaterials.2019.119311
Mahmoudzadeh A, Mohammadpour H, 2016, Tumor cell culture on collagen-chitosan scaffolds as three-dimensional tumor model: A suitable model for tumor studies. J Food Drug Anal, 24(3): 620–626. https://doi.org/10.1016/j.jfda.2016.02.008
Ananthanarayanan B, Kim Y, Kumar S, 2011, Elucidating the mechanobiology of malignant brain tumors using a brain matrix-mimetic hyaluronic acid hydrogel platform. Biomaterials, 32(31): 7913–7923. https://doi.org/10.1016/j.biomaterials.2011.07.005
Kwak BS, Choi W, Jeon J-W, et al., 2018, In vitro 3D skin model using gelatin methacrylate hydrogel. J Ind Eng Chem, 66: 254–261. https://doi.org/10.1016/j.jiec.2018.05.037
Pulaski BA, S. O-R, 2000, Mouse 4T1 breast tumor model. Curr Protoc Immunol, 39(1): 1–16. https://doi.org/10.1002/0471142735.im2002s39
Gregorio AC, Fonseca NA, Moura V, et al., 2016, Inoculated cell density as a determinant factor of the growth dynamics and metastatic efficiency of a breast cancer murine model. PLoS One, 11(11): e0165817. https://doi.org/10.1371/journal.pone.0165817
Keklikoglou I, Cianciaruso C, Guc E, et al., 2019, Chemotherapy elicits pro-metastatic extracellular vesicles in breast cancer models. Nat Cell Biol, 21(2): 190–202. https://doi.org/10.1038/s41556-018-0256-3