Optimized vascular network by stereolithography for tissue engineered skin
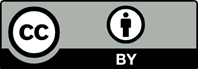
This paper demonstrates the essential and efficient methods to design, and fabricate optimal vascular network for tissue engineering structures based on their physiological conditions. Comprehensive physiological requirements in both micro and macro scales were considered in developing the optimisation design for complex vascular vessels. The optimised design was then manufactured by stereolithography process using materials that are biocompatible, elastic and surface bio-coatable. The materials are self-developed photocurable resin consist of BPA-ethoxylated-diacrylate, lauryl acrylate and isobornylacrylate with Irgacure® 184, the photoinitiator. The optimised vascular vessel offers many advantages: 1) it provides the maximum nutrient supply; 2) it minimises the recirculation areas and 3) it allows the wall shear stress on the vessel in a healthy range. The stereolithography manufactured vascular vessels were then embedded in the hydrogel seeded with cells. The results of in vitro studies show that the optimised vascular network has the lowest cell death rate compared with a pure hydrogel scaffold and a hydrogel scaffold embedded within a single tube in day seven. Consequently, these design and manufacture routes were shown to be viable for exploring and developing a high range complex and specialised artificial vascular networks.
[1]Ng WL, Qi JTZ, Yeong WY, et al., 2018, Proof-of-concept: 3D bioprinting of pigmented human skin constructs. Biofabrication, 10(2): 025005. https://doi.org/10.1088/1758-5090/aa9e1e
[2]NgWL, Wang S, Yeong WY, et al., 2016, Skin Bioprinting: Impending Reality or Fantasy? Trends Biotechnol, 34(9): 689–699. https://doi.org/10.1016/j.tibtech.2016.04.006
[3]Rose FR, Oreffo RO, 2002, Bone Tissue Engineering: Hope vs Hype. Biochem Biophys Res Commun, 292(1): 1–7. https://doi.org/10.1006/bbrc.2002.6519
[4]Radisc M, Yang L, Boublik J, et al., 2004, Medium perfusion enables engineering of compact and contractile cardiac tissue. Am J Physiol Heart Circ Physiol, 286(2): H507–H516. https://doi.org/10.1152/ajpheart.00171.2003
[5]Hoch E, Tovar G, Borchers K, 2014, Bioprinting of artificial blood vessels: current approaches towards a demanding goal. Eur J Cardiothorac Surg, 46(5): 767–778. https://doi.org/10.1093/ejcts/ezu242
[6]Kannan RY, Salacinski HJ, Sales K, et al., 2005, The roles of tissue engineering and vascularisation in the development of micro-vascular networks: A review. Biomaterials, 26(14): 1857–1875. https://doi.org/10.1016/j.biomaterials.2004.07.006
[7]Patrick Jr CW, 2000, Adipose tissue engineering: The future of breast and soft tissue reconstruction following tumor resection. Semin Surg Oncol, vol.19(3): 302–311.
[8]Kamel RA, Ong JF, Eriksson E, et al., 2013, Tissue Engineering of Skin. J Am Coll Surg, 217(3): 533–555. https://doi.org/10.1016/j.jamcollsurg.2013.03.027
[9]Zdrahala R, 1996, Small caliber vascular grafts. Part II: Polyurethanes revisited. J Biomater Appl, 11(1): 37–61. https://doi.org/10.1177/088532829601100102
[10]Ng WL, Lee JM, Yeong WY, et al., 2017, Microvalve-based bioprinting – process, bio-inks and applications. Biomater Sci, 5(4): 632. https://doi.org/10.1039/c6bm00861e
[11]Melchels FPW, Domingos MAN, Klein TJ, et al., 2012, Additive manufacturing of tissues and organs. Prog Polym Sci, 37(8): 1079–1104. http://dx.doi.org/10.1016/j.progpolymsci.2011.11.007
[12]Miller JS, Stevens KR, Yang MT, et al., 2012, Rapid casting of patterned vascular networks for perfusable engineered three-dimensional tissues. Nat Mater, 11(9): 768–774. https://doi.org/10.1038/nmat3357
[13]Kolesky D, Truby R, Gladman S, et al., 2014, 3D Bioprinting of Vascularized, Heterogeneous Cell-Laden Tissue Constructs. Adv Mater, 26(19): 3124–3130. https://doi.org/10.1002/adma.201305506
[14]Wu W, DeConinck A, Lewis J, 2011, Omnidirectional Printing of 3D Microvascular Networks. Adv Mater, 23(24): H178–H183. https://doi.org/10.1002/adma.201004625
[15]Kucukgul C, Ozler B, Karakas HE, et al., 2013, 3D hybrid bioprinting of macrovascular structures. Procedia Eng, 59: 183–192. https://doi.org/10.1016/j.proeng.2013.05.109
[16]Wegener M, Burkhard E, Novosel E, et al., 2012, Soft polymers for building up small and smallest blood supplying system by stereolithography. J Funct Biomater, 3(2): 257–268. https://doi.org/10.3390/jfb3020257
[17]Hinton T, Jallerat Q, Palchesko R, 2015, Three-dimensional printing of complex biological structures by freeform reversible embedding of suspended hydrogels. Sci Adv, 1(9): e1500758. https://doi.org/10.1126/sciadv.1500758
[18]Han X, Bibb R, Harris R, 2015, Design of bifurcation junctions in artificial vascular vessels additively manufactured for skin tissue engineering. J Vis Lang Comput, 28: 238-249. https://doi.org/10.1016/j.jvlc.2014.12.005
[19]Han X, Bibb R, Harris R, 2016, Engineering Design of Artificial Vascular Junctions for 3D Printing. Biofabrication, 8(2): 025018. https://doi.org/10.1088/1758-5090/8/2/025018
[20]Kohler U, Marshall I, Robertson MB, et al., 2001, MRI measurement of wall shear stress vectors in bifurcation models and comparison with CFD predictions. J Magn Reson Imaging, 14(5): 563–573.
[21]Marshall I, Zhao S, Papathanasopoulou P, et al., 2004, MRI and CFD studies of pulsatile flow in healthy and stenosed carotid bifurcation models. J Biomech, 37(5): 679–687. https://doi.org/10.1016/j.jbiomech.2003.09.032
[22]Ravensbergen J, Krijger J, Hillen B, et al., 1995, Merging flows in an arterial confluence: the vertebra-basilar junction. J Fluid Mech, 304: 119–141. https://doi.org/10.1017/S0022112095004368
[23]Ravensbergen J, Krijger JKB, Verdaasdonk AL, et al., 1997, The influence of the blunting of the apex on the flow in a Vertebro-Basilar junction model. J Biomech Eng, 119(2): 195–205.
[24]Papaioannou TG, Stefanadis C, 2004, Vascular wall shear stress: Basic principles and methods. Hellenic J Cardiol, 46(1): 9–15.
[25]Caro CG, 2009, Discovery of the role of wall shear in atherosclerosis. Arterioscler Thromb Vasc Biol, 29(2): 158–161. https://doi.org/10.1161/ATVBAHA.108.166736
[26]Coppola G, Caro CG, 2009, Arterial geometry, flow pattern, wall shear and mass transport: Potential physiological significance. J R Soc Interface, 6(35): 1–10. https://doi.org/10.1098/rsif.2008.0417
[27]Liu G, Wu J, Ghista DNH, et al., 2015, Hemodynamic characterization of transient blood flow in right coronary arteries with varying curvature and side-branch bifurcation angles. Comput Biol Med, 64: 117–126. https://doi.org/10.1016/j.compbiomed.2015.06.009
[28]Edelman ER, 1999, Vascular Tissue Engineering: Designer Arteries. Circ Res, 85(12): 1115–1117.
[29]Friedman MH, Deters OJ, Mark FF, et al., 1983, Arterial geometry affects hemodynamics: a potential risk factor for atherogenesis. Atherosclerosis, 46(2): 225–231.
[30]Peng CN, Wang XQ, Xian ZC, et al., 2016, The Impact of the Geometric Characteristics on the Hemodynamics in the Stenotic Coronary Artery. PLoS One, 11(6): e0157490. https://dx.doi.org/10.1371%2Fjournal.pone.0157490
[31]Rabinovitz RS, Levesque MJ, Nerem RM, 1987, Effects of branching angle in the left main coronary bifurcation. Circulation, IV-387.
[32]Murray C, 1926, The physiological principle of minimum work. I. the vascular system and the cost of the blood volume. Proc Natl Acad Sci USA, 12(3): 207–214.
[33]Murray C, 1926, The physiological principle of minimum work: II Oxygen exchange in capillaries. Proc Natl Acad Sci USA, 12(5): 299–304.
[34]Zamir M, 1976, Optimality principles in arterial branching. J Theor Biol, 62(1): 227–251. https://doi.org/10.1016/0022-5193(76)90058-8
[35]Schreiner W, Karch R, Neumann M, et al., 2006, Optimized arterial trees supplying hollow organs. Med Eng Phys, 28(5): 416–429. https://doi.org/10.1016/j.medengphy.2005.07.019
[36]Kretoeski M, Rolland Y, Bezy-Wendling J, et al., 2003, Fast algorithm for 3-D vascular tree modeling. Computer Methods and Programs in Biomedicine, 70(2): 129–136.
[37]Kassab GS, Rider CA, Tang NJ, et al., 1993, Morphometry of pig coronary arterial trees. Am J Physiol, 265(1 Pt 2): H350–365. https://doi.org/10.1152/ajpheart.1993.265.1.H350
[38]Kamiya A, Togawa T, 1972, Optimal branching structure of the vascular tree. Bull Math Biophys, 34(4): 431–438.
[39]Regittnig W, Ellmerer M, Fauler G, et al., 2003, Assessment of transcapillary glucose exchange in human skeletal muscle and adipose tissue. Am J Physiol Endocrinol Metab, 285(2): E241–E251. https://doi.org/10.1152/ajpendo.00351.2002
[40]Khamassi J, Bierwisch C, Pelz P, 2016, Geometry optimization of branchings in vascular networks. Phys Rev E, 93(6): 062408. https://doi.org/10.1103/PhysRevE.93.062408
[41]Gebhardt A, 2013, Generative Fertigungsverfahren: Additive Manufacturing und 3D Drucken für Prototyping–Tooling–Produktion. Carl Hanser Verlag, München. Available from: https://www.hanser-elibrary.com/doi/pdf/10.3139/9783446436527.fm
[42]Gibson I, Rosen DW, Stucker B, 2010, Additive manufacturing technologies: Rapid prototyping to direct digital manufacturing. Springer, US. https://doi.org/10.1007/978-1-4419-1120-9
[43]Maruo S, Ikuta K, 2002, Submicron stereolithography for the production of freely movable mechanisms by using single-photon polymerization. Sens Actuators A Phys, 100(1): 70–76. https://doi.org/10.1016/S0924-4247(02)00043-2
[44]Engelhardt S, Hu Y, Seiler N, et al., 2011, 3D-microfabrication of polymer-protein hybrid structures with a q-switched microlaser. J Laser Micro Nanoen, 6(1): 54–58. http://dx.doi.org/10.2961/jlmn.2011.01.0012
[45]Young S, 2014, Blut für Kunstorgane. Available from: https://www.heise.de/tr/artikel/Blut-fuer-Kunstorgane-2156687.html
[46]Engelhardt S, Hoch E, Borchers K, et al., 2011, Fabrication of 2D protein microstructures and 3D polymer–protein hybrid microstructures by two-photon polymerization. Biofabrication, 3(2): 025003. https://doi.org/10.1088/1758-5082/3/2/025003
[47]Labana S, 1977, Chemistry and properties of crosslinked polymers. Academic Press, New York.