Deformation monitoring and stress distribution prediction of 3D-printed hydroxyapatite scaffolds
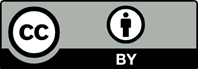
Hydroxyapatite (HAP) scaffolds created through biological three-dimensional (3D) printing have been extensively utilized in bone tissue engineering. However, clinical outcomes remain unsatisfactory. Stress-induced fractures are a primary cause of failure in clinical bone implant repairs. Finite element analysis (FEA) can predict stress distribution within scaffolds under load, aiding in the identification of stress concentrations. Nevertheless, limitations in the printing fidelity of bioinks lead to discrepancies between the printed scaffold structure and the theoretical design, resulting in inaccurate predictions. This study introduced an effective approach for predicting the mechanical properties and internal stress distribution of printed scaffolds. HAP scaffolds with varying filling angles (90º, 60º, 45º) were constructed through 3D printing. These scaffolds were analyzed using a combination of optical coherence tomography (P-OCT) scanning and micro-computed tomography (micro-CT) imaging, integrated with FEA, to enhance the accuracy of stress distribution predictions. The findings demonstrated that the mechanical strength of scaffolds predicted by the reconstructed model was closer to experimental values compared to predictions made by commonly used theoretical models (improved from 55% to 78%). During actual testing, stress concentration sites predicted by the reconstructed model were the first to exhibit fragmentation, validating the accuracy of stress distribution predictions. Moreover, scaffolds printed at different infill angles displayed varying degrees of distortion. Scaffolds printed at a 90º angle displayed the highest fidelity with the fewest defects, and their stress distributions under different conditions were well-correlated. The method proposed in this study facilitates more accurate prediction and evaluation of scaffold performance early in development, enabling the selection of scaffolds with suitable mechanical properties, thereby reducing testing cycles and improving safety in bone defect repair.
- Schemitsch EH. Size matters: defining critical in bone defect size! J Orthop Trauma. 2017;31(Suppl 5):S20-S22. doi: 10.1097/BOT.0000000000000978
- Kang Y, Xu C, Meng LA, et al. Exosome-functionalized magnesium-organic framework-based scaffold with osteogenic, angiogenic and anti-inflammatory properties foraccelerated bone regeneration. Bioact. Mater. 2022; 18:26-41. doi: 10.1016/j.bioactmat.2022.02.012
- Turnbull G, Clarke J, Picard F, et al. 3D bioactive composite scaffolds for bone tissue engineering. Bioact Mater. 2018;3(3):278-314. doi: 10.1016/j.bioactmat.2017.10.001
- Anjum S, Rahman F, Pandey P, et al. Electrospun biomimetic nanofibrous scaffolds: a promising prospect for bone tissue engineering and regenerative medicine. Int J Mol Sci. 2022;23(16):9206. doi: 10.3390/ijms23169206
- Huang L, Zhang J, Liu X, et al. L-Arginine/nanofish bone nanocomplex enhances bone regeneration via antioxidant activities and osteoimmunomodulatory properties. Chinese Chem Lett. 2021;32(1):234-238. doi: 10.1016/j.cclet.2020.11.046
- Liu X, Ma PX. Polymeric scaffolds for bone tissue engineering. Ann Biomed Eng. 2004;32(3):9622-9629. doi: 10.1023/b:abme.0000017544.36001.8e
- Pareek A, Reardon PJ, Macalena JA, et al. Osteochondral autograft transfer versus microfracture in the knee: a meta-analysis of prospective comparative studies at midterm. Arthroscopy. 2016;32(10):2118-2130. doi: 10.1016/j.arthro.2016.05.038
- Zhou S, Jung S, Hwang J. Mechanical analysis of femoral stress-riser fractures. Clin Biomech. 2019;63:10-15. doi: 10.1016/j.clinbiomech.2019.02.004
- Huo J, Dérand P, Rnnar LE, et al. Failure location prediction by finite element analysis for an additive manufactured mandible implant. Med Eng Phys. 2015;37(9):862-869. doi: 10.1016/j.medengphy.2015.06.001
- Dong J, Li Y, Lin P, et al. Solvent-cast 3D printing of magnesium scaffolds. Acta Biomater. 2020;114:497-514. doi: 10.1016/j.actbio.2020.08.002
- Barbetta A, Costantini M. Gas Foaming Technologies for 3D Scaffold Engineering. Woodhead Publishing; 2018:127-149. doi: 10.1016/b978-0-08-100979-6.00006-9
- Kordjamshidi A, Saber-Samandari S, Nejad MG, Khandan A. Preparation of novel porous calcium silicate scaffold loaded by celecoxib drug using freeze drying technique: fabrication, characterization and simulation. Ceram Int. 2019;45(11):14126-14135. doi: 10.1016/j.ceramint.2019.04.113
- Dong Z, Cui H, Zhang H, et al. 3D printing of inherently nanoporous polymers via polymerization-induced phase separation. Nat Commun. 2021;12(1):247. doi: 10.1038/s41467-020-20498-1
- Maharjan B, Kaliaanagounder VK, Jang SR, et al. In-situ polymerized polypyrrole nanoparticles immobilized poly(ε-caprolactone) electrospun conductive scaffolds for bone tissue engineering. Mater Sci Eng C Mater Biol Appl. 2020;114:111056. doi: 10.1016/j.msec.2020.111056
- Shuai C, Yang W, Feng P, Peng S, Pan H. Accelerated degradation of HAP/PLLA bone scaffold by PGA blending facilitates bioactivity and osteoconductivity. Bioact Mater. 2021;6(2):490-502. doi: 10.1016/j.bioactmat.2020.09.001
- Su J, Hua S, Chen A, et al. Three-dimensional printing of gyroid-structured composite bioceramic scaffolds with tuneable degradability. Biomater Adv. 2021;133:112595. doi: 10.1016/j.msec.2021.112595
- Xiao J, Xue H, Qian Z, et al. Jumbo bionic trabecular metal acetabular cups improve cup stability during acetabular bone defect reconstruction:a finite element analysis study. J Bionic Eng. 2023;20(6):2814-2825. doi: 10.1007/s42235-023-00413-2
- Liu L, Liu C, Deng C, et al. Design and performance analysis of 3D-printed stiffness gradient femoral scaffold. J Orthop Surg Res. 2023;18(1):120. doi: 10.1186/s13018-023-03612-z
- Pang S, Wu D, Gurlo A, Kurreck J, Hanaor DAH. Additive manufacturing and performance of bioceramic scaffolds with different hollow strut geometries. Biofabrication 2023;15(2):025011. doi: 10.1088/1758-5090/acb387
- Hao YL, Li S-J, Yang R. Biomedical titanium alloys and their additive manufacturing. Rare Metals. 2016;35(009): 661-671. doi: 10.1007/s12598-016-0793-5
- Melenka GW, Schofield JS,Dawson MR, Carey JP. Evaluation of dimensional accuracy and material properties of the MakerBot 3D desktop printer. Rapid Prototyping J. 2015;21(5):618-627. doi: 10.1108/rpj-09-2013-0093
- Sharma R, Singh R, Penna R, Fraternali F. Investigations for mechanical properties of Hap, PVC and PP based 3D porous structures obtained through biocompatible FDM filaments. Compos Part B-Eng. 2018;132:237-243. doi: 10.1016/j.compositesb.2017.08.021
- Muhammad A, Ali MA, Shanono IH. Fatigue and harmonic analysis of a diesel engine crankshaft using ANSYS[C]// iMEC-APCOMS 2019: Proceedings of the 4th International Manufacturing Engineering Conference and The 5th Asia Pacific Conference on Manufacturing Systems. Springer Singapore; 2020:371-376. doi: 10.1007/978-981-15-0950-6_56
- Liu H, Ahlinder A, Yassin MA, et al. Computational and experimental characterization of 3D-printed PCL structures toward the design of soft biological tissue scaffolds. Mater Design. 2020;188:108488. doi: 10.1016/j.matdes.2020.108488
- Soufivand AA, Abolfathi N, Hashemi A, Lee SJ. Prediction of mechanical behavior of 3D bioprinted tissue-engineered scaffolds using finite element method (FEM) analysis. Addit Manuf. 2020;33(8):101181. doi: 10.1016/j.addma.2020.101181
- de Galarreta SR, Jeffers JRT, Ghouse S. A validated finite element analysis procedure for porous structures. Mater Design. 2020;189:108546. doi: 10.1016/j.matdes.2020.108546
- Zhou J, Huang H, Wang LJ, et al. Stable mechanical fixation in a bionic osteochondral scaffold considering bone growth. Rare Metals. 2022;41(8):2711-2718. doi: 10.1007/s12598-022-02000-6
- Mohol SS, Kumar M, Sharma V. PLA-based nature-inspired architecture for bone scaffolds: a finite element analysis. Comput Biol Med. 2023;163:107163. doi: 10.1016/j.compbiomed.2023.107163
- Scocozza F, Gravina GMD, Bari E, et al. Prediction of the mechanical response of a 3D (bio)printed hybrid scaffold for improving bone tissue regeneration by structural finite element analysis. J Mech Behav Biomed Mater. 2023;142:105822. doi: 10.1016/j.jmbbm.2023.105822
- Zhang B, Guo L, Chen H, Ventikos Y, Narayan RJ, Huang J. Finite element evaluations of the mechanical properties of polycaprolactone/hydroxyapatite scaffolds by direct ink writing: effects of pore geometry. J Mech Behav Biomed Mater. 2020;104:103665. doi: 10.1016/j.jmbbm.2020.103665
- Yunsheng D, Hui X, Jie W, et al. Sustained release silicon from 3D bioprinting scaffold using silk/gelatin inks to promote osteogenesis. Int J Biol Macromol. 2023;234:123659. doi: 10.1016/j.ijbiomac.2023.123659
- Yang S, Wang L, Chen Q, Xu M. J. A. M. In situ process monitoring and automated multi-parameter evaluation using optical coherence tomography during extrusion-based bioprinting. Addit Manuf. 2021;47:102251. doi: 10.1016/j.addma.2021.102251
- Yang S,Chen Q, Wang L, Xu M. In situ defect detection and feedback control with three-dimensional extrusion-based bioprinter-associated optical coherence tomography. Int J Bioprint. 2023;9(1):624. doi: 10.18063/ijb.v9i1.624
- Suo H,Chen Y, Liu J, Wang L, Xu M. 3D printing of biphasic osteochondral scaffold with sintered hydroxyapatite and polycaprolactone. J Mater Sci. 2021;56:16623-16633. doi: 10.1007/s10853-021-06229-x
- Keaveny TM, Morgan EF, Niebur GL, Yeh OC. Biomechanics of trabecular bone. Annu Rev Biomed Eng. 2001;3(1): 307-333. doi: 10.1146/annurev.bioeng.3.1.307
- Collins MN, Ren G, Young K, et al. Scaffold fabrication technologies and structure/function properties in bone tissue engineering. Adv Funct Mater. 2021;31(21):2010609. doi: 10.1002/adfm.202010609
- Lee JS, Cha HD, Shim JH, et al. Effect of pore architecture and stacking direction on mechanical properties of solid freeform fabrication‐based scaffold for bone tissue engineering. J Biomed Mater Res A. 2012;100A(7): 1846-1853. doi: 10.1002/jbm.a.34149