3D-Printed mesoporous bioglass/ polycaprolactone scaffolds induce macrophage polarization toward M2 phenotype and immunomodulates osteogenic differentiation of BMSCs
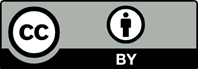
Bioceramic composite polycaprolactone (PCL) scaffolds are widely used in bone defect repair studies. Among them, bioactive glass (BG) is considered an excellent bone-based repair material due to its unique inorganic amorphous structure, bioactivity, and osseointegration properties. However, the dense pores and low specific surface area of ordinary BGs and mesoporous BGs limit the mechanical properties and bioactivity of the overall scaffolds, and it is often necessary to increase the proportion of BGs to offset these shortcomings. Here, we prepared highly active dendritic mesoporous structured bioactive glass (MBG) with a high specific surface area (457.14 m2/g) and pore volume (1.38 cm3/g) by sol-gel method. PCL scaffolds containing different percentages of MBG were prepared by three-dimensional printing technology, and the physicochemical and immunomodulatory osteogenic properties were investigated. The results showed that the low-concentration MBG/ PCL scaffolds with 10% content (10MBG/PCL) possessed the highest compressive strength (about two times that of pure PCL scaffolds) and excellent in vitro immunomodulatory osteogenic properties. Finally, 10MBG/PCL was selected for further exploration to investigate the effects of different fiber diameters (F300, F500, F800) and pore sizes (P200, P500, P800) on the scaffolds performance. Ultimately, we found that the 10MBG/PCL scaffolds with fiber diameter and pore size of 500 μm had high osteogenic potential, significantly induced macrophage polarization toward the M2 phenotype, and downregulated the expression of inflammatory genes and that this group was the most capable of mediating macrophage polarization and thus inducing the osteogenic differentiation of rat bone marrow mesenchymal stem cells (BMSCs) to form an immune microenvironment conducive to osteogenesis. This study is a step forward in the exploration of the performance of BG composite PCL scaffolds and provides a new idea for the development of bone graft materials.
- Ye S, Seo K-B, Park B-H, et al. Comparison of the osteogenic potential of bone dust and iliac bone chip. Spine J. 2013;13(11):1659-1666. doi: 10.1016/j.spinee.2013.06.012
- Zhang J, Jiang Y, Shang, et al. Biodegradable metals for bone defect repair: A systematic review and meta-analysis based on animal studies. Bioact Mater. 2021;6(11): 4027-4052. doi: 10.1016/j.bioactmat.2021.03.035
- Khodakaram-Tafti A, Mehrabani D, Shaterzadeh-Yazdi H, Zamiri B, Omidi, M. Tissue engineering in maxillary bone defects. World J Plast Surg. 2018;7(1):3-11.
- Turnbull G, Clarke J, Picard F, et al. 3D bioactive composite scaffolds for bone tissue engineering. Bioact Mater. 2018;3(3):278-314. doi: 10.1016/j.bioactmat.2017.10.001
- Siddiqui N, Asawa S, Birru B, Baadhe R, Rao S. PCL-based composite scaffold matrices for tissue engineering applications. Mol Biotechnol. 2018;60(7):506-532. doi: 10.1007/s12033-018-0084-5
- Gharibshahian M, Salehi M, Beheshtizadeh N, et al. Recent advances on 3D-printed PCL-based composite scaffolds for bone tissue engineering. Front Bioeng Biotechnol. 2023;11:1168504. doi: 10.3389/fbioe.2023.1168504
- Jones JR. Reprint of: review of bioactive glass: from Hench to hybrids. Acta Biomater. 2015;23(Suppl 1):S53-S82. doi: 10.1016/j.actbio.2015.07.019
- Hench LL, Polak JM. Third-generation biomedical materials. Science. 2002;295(5557):1014. doi: 10.1126/science.1067404
- Kokubo T, Kim HM, Kawashita M. Novel bioactive materials with different mechanical properties. Biomaterials. 2003;24(13):2161-2175. doi: 10.1016/s0142-9612(03)00044-9
- Skallevold HE, Rokaya D, Khurshid Z, Zafar MS. Bioactive glass applications in dentistry. Int J Mol Sci. 2019;20(23). doi: 10.3390/ijms20235960
- Schumacher M, Habibovic P, van Rijt S. Mesoporous bioactive glass composition effects on degradation and bioactivity. Bioact Mater. 2021;6(7):1921-1931. doi: 10.1016/j.bioactmat.2020.12.007
- Zheng K, Boccaccini AR. Sol-gel processing of bioactive glass nanoparticles: a review. Adv Colloid Interface Sci. 2017;249:363-373. doi: 10.1016/j.cis.2017.03.008
- El-Fiqi A, Mandakhbayar N, Jo SB, et al. Nanotherapeutics for regeneration of degenerated tissue infected by bacteria through the multiple delivery of bioactive ions and growth factor with antibacterial/angiogenic and osteogenic/ odontogenic capacity. Bioact Mater. 2021;6(1):123-136. doi: 10.1016/j.bioactmat.2020.07.010
- Xin T, Gu Y, Cheng R, et al. Inorganic strengthened hydrogel membrane as regenerative periosteum. Acs Appl Mater Interfaces. 2017;9(47):41168-41180. doi: 10.1021/acsami.7b13167
- Zhao H, Wang X, Jin A, et al. Reducing relapse and accelerating osteogenesis in rapid maxillary expansion using an injectable mesoporous bioactive glass/fibrin glue composite hydrogel. Bioact Mater. 2022;18:507-525. doi: 10.1016/j.bioactmat.2022.03.001
- Wang C, Meng C, Zhang Z, Zhu Q. 3D printing of polycaprolactone/bioactive glass composite scaffolds for in situ bone repair. Ceram Int. 2022;48(6):7491-7499. doi: 10.1016/j.ceramint.2021.11.293
- Huang W, Cai X, Xiao C, Song W, Yin H, Xu W. Surface micropatterning of 3D printed PCL scaffolds promotes osteogenic differentiation of BMSCs and regulates macrophage M2 polarization. Heliyon 2024;10(5):e26621-e26621. doi: 10.1016/j.heliyon.2024.e26621
- Arron JR, Choi Y. Osteoimmunology - bone versus immune system. Nature. 2000;408(6812):535-536. doi: 10.1038/35046196
- Yang N, Liu Y. The role of the immune microenvironment in bone regeneration. Int J Med Sci. 2021;18(16):3697-3707. doi: 10.7150/ijms.61080
- Wu Z, Bai J, Ge G, et al. Regulating macrophage polarization in high glucose microenvironment using lithium-modified bioglass-hydrogel for diabetic bone regeneration. Adv Healthc Mater. 2022;11(13). doi: 10.1002/adhm.202200298
- Feito MJ, Casarrubios L, Onaderra M, et al. Response of RAW 264.7 and J774A.1 macrophages to particles and nanoparticles of a mesoporous bioactive glass: a comparative study. Colloids Surf B. 2021;208. doi: 10.1016/j.colsurfb.2021.112110
- Xu H, Zhu Y, Hsiao AW-T, et al. Bioactive glass-elicited stem cell-derived extracellular vesicles regulate M2 macrophage polarization and angiogenesis to improve tendon regeneration and functional recovery. Biomaterials. 2023;294. doi: 10.1016/j.biomaterials.2023.121998
- Zheng K, Niu W, Lei B, Boccaccini AR. Immunomodulatory bioactive glasses for tissue regeneration. Acta Biomater. 2021;133:168-186. doi: 10.1016/j.actbio.2021.08.023
- Gomez-Cerezo N, Casarrubios L, Morales I, et al. Effects of a mesoporous bioactive glass on osteoblasts, osteoclasts and macrophages. J Colloid Interface Sci. 2018;528: 309-320. doi: 10.1016/j.jcis.2018.05.099
- Huang Y, Wu C, Zhang X, Chang J, Dai K. Regulation of immune response by bioactive ions released from silicate bioceramics for bone regeneration. Acta Biomater. 2018;66:81-92. doi: 10.1016/j.actbio.2017.08.044
- Singh RP, Ramarao P. Accumulated polymer degradation products as effector molecules in cytotoxicity of polymeric nanoparticles. Toxicol Sci. 2013;136(1):131-143. doi: 10.1093/toxsci/kft179
- Wang X, Zachman AL, Chun YW, Shen F-W, Hwang Y-S, Sung H-J. Polymeric stent materials dysregulate macrophage and endothelial cell functions: implications for coronary artery stent. Int J Cardiol. 2014;174(3):688-695. doi: 10.1016/j.ijcard.2014.04.228
- Wang Z, Cui Y, Wang J, et al. The effect of thick fibers and large pores of electrospun poly(ε-caprolactone) vascular grafts on macrophage polarization and arterial regeneration. Biomaterials. 2014;35(22):5700-5710. doi: 10.1016/j.biomaterials.2014.03.078
- Abebayehu D, Spence A, Boyan BD, Schwartz Z, Ryan JJ, McClure MJ. Galectin-1 promotes an M2 macrophage response to polydioxanone scaffolds. J Biomed Mater Res Part A. 2017;105(9):2562-2571. doi: 10.1002/jbm.a.36113
- Zhu G, Zhang R, Xie Q, et al. Shish-kebab structure fiber with nano and micro diameter regulate macrophage polarization for anti-inflammatory and bone differentiation. Mater Today Bio. 2023;23. doi: 10.1016/j.mtbio.2023.100880
- Wang Y, Liao T, Shi M, Liu C, Chen X. Facile synthesis and in vitro bioactivity of radial mesoporous bioactive glasses. Mater Lett. 2017;206:205-209. doi: 10.1016/j.matlet.2017.07.021
- Chaudhary S, Ghosal D, Tripathi P, Kumar S. Cellular metabolism: a link connecting cellular behaviour with the physiochemical properties of biomaterials for bone tissue engineering. Biomater Sci. 2023;11(7):2277-2291. doi: 10.1039/d2bm01410f
- Lewallen EA, Trousdale WH, Thaler R, et al. Surface roughness of titanium orthopedic implants alters thebiological phenotype of human mesenchymal stromal cells. Tissue Eng Part A. 2021;27(23-24):1503-1516. doi: 10.1089/ten.tea.2020.0369
- Yuan B, Zhou S-y, Chen X-s. Rapid prototyping technology and its application in bone tissue engineering. J Zhejiang Univ Sci B. 2017;18(4):303-315. doi: 10.1631/jzus.B1600118
- van der Heide D, Cidonio G, Stoddart MJ, D’Este M. 3D printing of inorganic-biopolymer composites for bone regeneration. Biofabrication. 2022;14(4). doi: 10.1088/1758-5090/ac8cb2
- Garot C, Bettega G, Picart C. Additive manufacturing of material scaffolds for bone regeneration: toward application in the clinics. Adv Funct Mater. 2021;31(5). doi: 10.1002/adfm.202006967
- Feng Y, Zhu S, Mei D, et al. Application of 3D printing technology in bone tissue engineering: a review. Curr Drug Delivery. 2021;18(7):847-861. doi: 10.2174/1567201817999201113100322
- Ebrahimi S, Sipaut CS. The effect of liquid phase concentration on the setting time and compressive strength of hydroxyapatite/bioglass composite cement. Nanomaterials. 2021;11(10). doi: 10.3390/nano11102576
- Rupp F, Liang L, Geis-Gerstorfer J, Scheideler L, Huettig F. Surface characteristics of dental implants: a review. Dent Mater. 2018;34(1):40-57. doi: 10.1016/j.dental.2017.09.007
- Wang L, He S, Wu X, et al. Polyetheretherketone/nano-fluorohydroxyapatite composite with antimicrobial activity and osseointegration properties. Biomaterials. 2014;35(25):6758-6775. doi: 10.1016/j.biomaterials.2014.04.085
- Muecksch C, Urbassek HM. Accelerated molecular dynamics study of the effects of surface hydrophilicity on protein adsorption. Langmuir. 2016;32(36): 9156-9162. doi: 10.1021/acs.langmuir.6b02229
- Wang X, Molino BZ, Pitkanen S, et al. 3D scaffolds of polycaprolactone/copper-doped bioactive glass: architecture engineering with additive manufacturing and cellular assessments in a coculture of bone marrow stem cells and endothelial cells. Acs Biomater Sci Eng. 2019;5(9): 4496-4510. doi: 10.1021/acsbiomaterials.9b00105
- Zhou L, Fan L, Zhang F-M, et al. Hybrid gelatin/oxidized chondroitin sulfate hydrogels incorporating bioactive glass nanoparticles with enhanced mechanical properties, mineralization, and osteogenic differentiation. Bioact Mater. 2021;6(3):890-904. doi: 10.1016/j.bioactmat.2020.09.012
- Yazdimamaghani M, Razavi M, Vashaee D, Moharamzadeh K, Boccaccini AR, Tayebi L. Porous magnesium-based scaffolds for tissue engineering. Mater Sci Eng C Mater Biol Appl. 2017;71:1253-1266. doi: 10.1016/j.msec.2016.11.027
- Marrella A, Lee TY, Lee DH, et al. Engineering vascularized and innervated bone biomaterials for improved skeletal tissue regeneration. Mater Today. 2018;21(4):362-376. doi: 10.1016/j.mattod.2017.10.005
- Axpe E, Oyen ML. Applications of alginate-based bioinks in 3D bioprinting. Int J Mol Sci. 2016;17(12). doi: 10.3390/ijms17121976
- Fiocco L, Elsayed H, Badocco D, et al. Direct ink writing of silica-bonded calcite scaffolds from preceramic polymers and fillers. Biofabrication. 2017;9(2). doi: 10.1088/1758-5090/aa6c37
- Mastrogiacomo M, Scaglione S, Martinetti R, et al. Role of scaffold internal structure on in vivo bone formation in macroporous calcium phosphate bioceramics. Biomaterials. 2006;27(17):3230-3237. doi: 10.1016/j.biomaterials.2006.01.031
- Hayashi K, Yanagisawa T, Kishida R, Ishikawa K. Effects of scaffold shape on bone regeneration: tiny shape differences affect the entire system. Acs Nano. 2022;16(8):11755-11768. doi: 10.1021/acsnano.2c03776
- Gorustovich AA, Roether JA, Boccaccini AR. Effect of bioactive glasses on angiogenesis: a review of in vitro and in vivo evidences. Tissue Eng Part B Rev. 2010;16(2):199-207. doi: 10.1089/ten.teb.2009.0416
- Hench LL. Genetic design of bioactive glass. J Eur Ceram Soc. 2009;29(7):1257-1265. doi: 10.1016/j.jeurceramsoc.2008.08.002
- Ajita J, Saravanan S, Selvamurugan N. Effect of size of bioactive glass nanoparticles on mesenchymal stem cell proliferation for dental and orthopedic applications. Mater Sci Eng C Mater Biol Appl. 2015;53:142-149. doi: 10.1016/j.msec.2015.04.041
- El-Rashidy AA, Roether JA, Harhaus L, Kneser U, Boccaccini AR. Regenerating bone with bioactive glass scaffolds: a review of in vivo studies in bone defect models. Acta Biomater. 2017;62:1-28. doi: 10.1016/j.actbio.2017.08.030
- Sepulveda P, Jones JR, Hench LL. Characterization of melt-derived 45S5 and sol-gel-derived 58S bioactive glasses. J Biomed Mater Res. 2001;58(6):734-740. doi: 10.1002/jbm.10026
- Xie W, Fu X, Tang F, et al. Dose-dependent modulation effects of bioactive glass particles on macrophages and diabetic wound healing. J Mater Chem B. 2019;7(6):940-952. doi: 10.1039/c8tb02938e
- Gao Q, Xie C, Wang P, et al. 3D printed multi-scale scaffolds with ultrafine fibers for providing excellent biocompatibility. Mater Sci Eng C Mater Biol Appl. 2020;107. doi: 10.1016/j.msec.2019.110269
- Sanz M, Dahlin C, Apatzidou D, et al. Biomaterials and regenerative technologies used in bone regeneration in the craniomaxillofacial region: consensus report of group 2 of the 15th European Workshop on Periodontology on Bone Regeneration. J Clin Periodontol. 2019;46:82-91. doi: 10.1111/jcpe.13123
- Yedekci B, Tezcaner A, Yilmaz B, Demir T, Evis Z. 3D porous PCL-PEG-PCL/strontium, magnesium and boron multi-doped hydroxyapatite composite scaffolds for bone tissue engineering. J Mech Behav Biomed Mater. 2022;125. doi: 10.1016/j.jmbbm.2021.104941
- Swanson WB, Omi M, Zhang Z, et al. Macropore design of tissue engineering scaffolds regulates mesenchymal stem cell differentiation fate. Biomaterials. 2021;272. doi: 10.1016/j.biomaterials.2021.120769
- Wang C, Wu J, Liu L, et al. Improving osteoinduction and osteogenesis of Ti6Al4V alloy porous scaffold by regulating the pore structure. Front Chem. 2023;11. doi: 10.3389/fchem.2023.1190630
- Garg K, Pullen NA, Oskeritzian CA, Ryan JJ, Bowlin GL. Macrophage functional polarization (M1/M2) in response to varying fiber and pore dimensions of electrospun scaffolds. Biomaterials. 2013;34(18):4439-4451. doi: 10.1016/j.biomaterials.2013.02.065
- Horii T, Tsujimoto H, Hagiwara A, et al. Effects of fiber diameter and spacing size of an artificial scaffold on the in vivo cellular response and tissue remodeling. ACS Appl Biomater. 2021;4(9):6924-6936. doi: 10.1021/acsabm.1c00572
- Li W, Dai F, Zhang S, et al. Pore size of 3D-printed polycaprolactone/polyethylene glycol/hydroxyapatite scaffolds affects bone regeneration by modulating macrophage polarization and the foreign body response. Acs Appl Mater Interfaces. 2022;14(18):20693-20707. doi: 10.1021/acsami.2c02001
- Yang X, Gao J, Yang S, et al. Pore size-mediated macrophage M1 to M2 transition affects osseointegration of 3D-printed PEEK scaffolds. Int J Bioprint. 2023;9(5):128-144. doi: 10.18063/ijb.755