Advances in Filament Structure of 3D Bioprinted Biodegradable Bone Repair Scaffolds
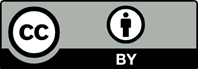
Conventional bone repair scaffolds can no longer meet the high standards and requirements of clinical applications in terms of preparation process and service performance. Studies have shown that the diversity of filament structures of implantable scaffolds is closely related to their overall properties (mechanical properties, degradation properties, and biological properties). To better elucidate the characteristics and advantages of different filament structures, this paper retrieves and summarizes the state of the art in the filament structure of the three-dimensional (3D) bioprinted biodegradable bone repair scaffolds, mainly including single-layer structure, double-layer structure, hollow structure, core-shell structure and bionic structures. The eximious performance of the novel scaffolds was discussed from different aspects (material composition, ink configuration, printing parameters, etc.). Besides, the additional functions of the current bone repair scaffold, such as chondrogenesis, angiogenesis, anti-bacteria, and anti-tumor, were also concluded. Finally, the paper prospects the future material selection, structural design, functional development, and performance optimization of bone repair scaffolds.
1. Lan L, Fei Y, Shi J, et al., 2017, In Situ Repair of Bone and Cartilage Defects Using 3D Scanning and 3D Printing. Sci Rep, 7:9416. https://doi.org/10.1038/s41598-017-10060-3
2. Annamalai RT, Hong X, Schott NG, et al., 2019, Injectable Osteogenic Microtissues Containing Mesenchymal Stromal Cells Conformally Fill and Repair Critical-Size Defects. Biomaterials, 208:32–44. https://doi.org/10.1016/j.biomaterials.2019.04.001
3. Zhang X, Li Y, Chen YE, et al., 2016, Cell-free 3D Scaffold with Two-stage Delivery of miRNA-26a to Regenerate Critical-sized Bone Defects. Nat Commun, 7:10376. https://doi.org/10.1038/ncomms10376
4. Schemitsch EH, 2017, Size Matters: Defining Critical in Bone Defect Size! J Orthop Trauma, 31:S20–2. https://doi.org/10.1097/BOT.0000000000000978
5. Lin KF, He S, Song Y, et al., 2016, Low-Temperature Additive Manufacturing of Biomimic Three-Dimensional Hydroxyapatite/Collagen Scaffolds for Bone Regeneration. ACS Appl Mater Interfaces, 8:6905–16. https://doi.org/10.1021/acsami.6b00815
6. Faldini C, Traina F, Perna F, et al., 2015, Surgical Treatment of Aseptic Forearm Nonunion with Plate and Opposite Bone Graft Strut. Autograft or Allograft? Int Orthop, 39:1343–9. https://doi.org/10.1007/s00264-015-2718-6
7. Arrington ED, Smith WJ, Chambers HG, et al., 1996, Complications of Iliac Crest Bone Graft Harvesting. Clin Orthop Relat Res, 329:300–9. https://doi.org/10.1243/09596518JSCE892
8. Lai Y, Cao H, Wang X, et al., 2018, Porous Composite Scaffold Incorporating Osteogenic Phytomolecule Icariin for Promoting Skeletal Regeneration in Challenging Osteonecrotic Bone in Rabbits. Biomaterials, 153:1–13. https://doi.org/10.1016/j.biomaterials.2017.10.025
9. Almubarak S, Nethercott H, Freeberg M, et al., 2016, Tissue Engineering Strategies for Promoting Vascularized Bone Regeneration. Bone, 83:197–209. https://doi.org/10.1016/j.bone.2015.11.011
10. Kim JA, Lim J, Naren R, et al., 2016, Effect of the Biodegradation Rate Controlled by Pore Structures in Magnesium Phosphate Ceramic Scaffolds on Bone Tissue Regeneration In Vivo. Acta Biomater, 44:155–67. https://doi.org/10.1016/j.actbio.2016.08.039
11. Williams DF, 2008, On the Mechanisms of Biocompatibility. Biomaterials, 29:2941–53. https://doi.org/10.1016/j.biomaterials.2008.04.023
12. Yao Y, Qin W, Xing B, et al., 2021, High performance Hydroxyapatite Ceramics and a Triply Periodic Minimum Surface Structure Fabricated by Digital Light Processing 3D printing. J Adv Ceramics, 10:39–48. https://doi.org/10.1007/s40145-020-0415-4
13. Rouwkema J, Rivior NC, Van CA, et al., 2008, Vascularization in Tissue Engineering. Trends Biotechnol, 26:434–41. https://doi.org/10.1016/j.tibtech.2008.04.009
14. Cao H, Kuboyama N, 2010, A Biodegradable Porous Composite Scaffold of PGA/beta-TCP for Bone Tissue Engineering. Bone, 46:386–95. https://doi.org/10.1016/j.bone.2009.09.031
15. Olszta M, Cheng X, Jee S, et al., 2007, Bone Structure and Formation: A New Perspective. Mater Sci Eng R Rep, 58:77–116. https://doi.org/10.1016/j.mser.2007.05.001
16. Bramfeldt H, Sabra G, Centis V, et al., 2010, Scaffold Vascularization: A Challenge for Three-Dimensional Tissue Engineering. CMC, 17:3944–67. https://doi.org/10.2174/092986710793205327
17. Jain RK, Au P, Tam J, et al., 2005, Engineering Vascularized Tissue. Nat Biotechnol, 23:821–3. https://doi.org/10.1038/nbt0705-821
18. Ma Y, Dai H, Huang X, et al., 2019, 3D Printing of Bioglass reinforced β-TCP Porous Bioceramic Scaffolds. J Mater, 54:10437–46. https://doi.org/10.1007/s10853-019-03632-3
19. Woodfield T, Malda J, Wijn JD, et al., 2004, Design of Porous Scaffolds for Cartilage Tissue Engineering Using a Threedimensional Fiber-deposition Technique. Biomaterials, 25:4149–61. https://doi.org/10.1016/j.biomaterials.2003.10.056
20. Hutmacher DW, 2001, Scaffold Design and Fabrication Technologies for Engineering Tissues State of the Art and Future Perspectives. J Biomater Sci Polym Ed, 12:107–24. https://doi.org/10.1163/156856201744489
21. ASTM F2792-12a, 2012, Standard Terminology for Additive Manufacturing Technologies. West Conshohocken, PA: ASTM International.
22. Shahrubudin N, Lee TC, Ramlan R, 2019, An Overview on 3D Printing Technology: Technological, Materials, and Applications. Proc Manufact, 35:1286–96. https://doi.org/10.1016/j.promfg.2019.06.089
23. Mahajan C, Cormier D, 2015, 3D Printing of Carbon Fiber Composites with Preferentially Aligend Fibers. Industrial and Systems Engineering Research Conference.
24. Yeong WY, Guo DG, 2020, 3D Printing of Carbon Fiber Composite: The Future of Composite Industry? Materials, 2:1361–3. https://doi.org/10.1016/j.matt.2020.05.010
25. Murphy SV, Coppi PD, Atala A, 2019, Opportunities and Challenges of Translational 3D Bioprinting. Nat Biomed Eng, 4:370–80. https://doi.org/10.1038/s41551-019-0471-7
26. Savage N, 2016, Technology: The Promise of Printing. Nature, 540:S56–7. https://doi.org/10.1038/540S56a
27. Boskey AL, 2015, Bone Composition: Relationship to Bone Fragility and Antiosteoporotic Drug Effects. Bonekey Rep, 4:710. https://doi.org/10.1038/bonekey.2015.79
28. Fu S, Zhu M, Zhu Y, 2019, Organosilicon Polymer-derived Ceramics: An Overview. J Adv Ceramics, 8:457–78. https://doi.org/10.1007/s40145-019-0335-3
29. Fahmy MD, Jazayeri HE, Razavi M, et al., 2016, Three-Dimensional Bioprinting Materials with Potential Application in Preprosthetic Surgery. J Prosthodont, 25:310–8. https://doi.org/10.1111/jopr.12431
30. Zhu W, Qu X, Zhu J, et al., 2017, Direct 3D Bioprinting of Prevascularized Tissue Constructs with Complex Microarchitecture. Biomaterials, 124:106–15. https://doi.org/10.1016/j.biomaterials.2017.01.042
31. Tang Z, Li X, Tan Y, et al., 2017, The Material and Biological Characteristics of Osteoinductive Calcium Phosphate Ceramics. Regener. Biomater. 5:43–59. https://doi.org/10.1093/rb/rbx024
32. Damien CJ, Parsons JR, 1991, Bone Graft Substitutes: A Review of Current Technology and Applications. Autumn (Fall). 2:187–208. https://doi.org/10.1002/jab.770020307
33. Oonishi H, 1991, Orthopaedic Applications of Hydroxyapatite. Biomaterials, 12:171–8. https://doi.org/10.1016/0142-9612(91)90196-H
34. Lowe B, Hardy JG, Walsh LJ, 2020, Optimizing Nanohydroxyapatite Nanocomposites for Bone Tissue Engineering. ACS Omega, 5:1–9. https://doi.org/10.1021/acsomega.9b02917
35. Desiraju GR, Hulliger J, 2001, Current Opinion in Solid State and Materials Science: Molecular Crystals and Materials. Curr Opin Solid State Mater Sci, 5:105–6. https://doi.org/10.1016/s1359-0286(01)00015-8
36. Li S, Liu Y, Zhang Q, et al., 2011, Microwave-assisted Coprecipitation Synthesis of High Purity β-tricalcium Phosphate Crystalline Powders. Mater Chem Phys, 129:1138–41. https://doi.org/10.1016/j.matchemphys.2011.05.075
37. Chen Z, Li Z, Li J, et al., 2018, 3D Printing of Ceramics: A Review. J Eur Ceramic Soc, 39:661–87. https://doi.org/10.1016/j.jeurceramsoc.2018.11.013
38. Jones JR, 2013, Review of Bioactive Glass: From Hench to Hybrids. Acta Biomater, 9:4457–86. https://doi.org/10.1016/j.actbio.2012.08.023
39. Fujishiro Y, Hench LL, Oonishi H, 1997, Quantitative Rates of In Vivo Bone Generation for Bioglass and Hydroxyapatite Particles as Bone Graft Substitute. J Mater Sci Mater Med, 8:649–52. https://doi.org/10.1023/A:1018527621356
40. Ducheyne P, 2010, Bioglass Coatings and Bioglass Composites as Implant Materials. J Biomed Mater Res Part A, 19:273–91. https://doi.org/10.1002/jbm.820190309
41. Li L, Hu H, Zhu Y, et al., 2019, 3D-printed Ternary SiO2CaO P2O5 bioglass-Ceramic Scaffolds with Tunable Compositions and Properties for Bone Regeneration. Ceramics Int, 45:10997–1005. https://doi.org/10.1016/j.ceramint.2019.02.183
42. Huang MH, Shen YF, Hsu TT, et al., 2016. Physical Characteristics, Antimicrobial and Odontogenesis Potentials of Calcium Silicate Cement Containing Hinokitiol. Mater Sci Eng C Mater Biol Appl, 65:1–8. https://doi.org/10.1016/j.msec.2016.04.016
43. Shie M, Chiang W, Chen I, et al., 2017, Synergistic Acceleration in the Osteogenic and Angiogenic Differentiation of Human Mesenchymal Stem Cells by Calcium Silicate-Graphene Composites. Mater Sci Eng C, 73:726–35. https://doi.org/10.1016/j.msec.2016.12.071
44. Bittner SM, Guo JL, Melchiorri A, et al., 2018, Three dimensional Printing of Multilayered Tissue Engineering Scaffolds. Mater Today, 21:861–74.
45. Sant S, Coutinho F, Gaharwar AK, et al., 2017, Self‐ Assembled Hydrogel Fiber Bundles from Oppositely Charged Polyelectrolytes Mimic Micro/Nanoscale Hierarchy of Collagen. Adv Funct Mater, 27:1606273. https://doi.org/10.1016/j.mattod.2018.02.006
46. Daniela L, Christoph M, Elke K, et al., 2016, Functionalization, Preparation and Use of Cell-laden Gelatin Methacryloyl based Hydrogels as Modular Tissue Culture Platforms. Nat Protoc Erec Res, 11:727–46. https://doi.org/10.1038/nprot.2016.037
47. Ying G, Jiang N, Maharjan S, et al., 2018, Bioprinting: Aqueous Two-Phase Emulsion Bioink-Enabled 3D Bioprinting of Porous Hydrogels. Adv Mater, 30:1870382. https://doi.org/10.1002/adma.201805460
48. Wang X, Jiang M, Zhou Z, et al., 2017, 3D Printing of Polymer Matrix Composites: A Review and Prospective. Comp Part B Eng, 110:442–58. https://doi.org/10.1016/j.compositesb.2016.11.034
49. Takezawa A, Kobashi M, 2017, Design Methodology for Porous Composites with Tunable Thermal Expansion Produced by Multi-Material Topology Optimization and Additive Manufacturing. Comp Part B Eng, 131:21–9. https://doi.org/10.1016/j.compositesb.2017.07.054
50. Li J, Xing R, Bai S, et al., 2019 Recent Advances of Self assembling Peptide-based Hydrogels for Biomedical Applications. Soft Matter, 30:1704–15. https://doi.org/10.1039/c8sm02573h
51. Pataky K, Braschler T, Negro A, et al., 2012, Micro drop Printing of Hydrogel Bioinks into 3D Tissue-Like Geometries. Adv Mater, 24:391–6. https://doi.org/10.1002/adma.201102800
52. Silva T, Moreira-Silva J, Marques A, et al., 2014, Marine Origin Collagens and its Potential Applications. Mar Drugs, 12:5881–901. https://doi.org/10.3390/md12125881
53. Hinton TJ, Jallerat Q, Palchesko RN, et al., 2015, Three dimensional Printing of Complex Biological Structures By Freeform Reversible Embedding of Suspended Hydrogels. Sci Adv, 1:e1500758. https://doi.org/10.1126/sciadv.1500758
54. Campos DF, Blaeser A, Korsten A, et al., 2015, The Stiffness and Structure of Three-dimensional Printed Hydrogels Direct the Differentiation of Mesenchymal Stromal Cells toward Adipogenic and Osteogenic Lineages. Tissue Eng Part A, 21:740–56. https://doi.org/10.1089/ten.TEA.2014.0231
55. Shim JH, Jang KM, Hahn SK, et al., 2016, Three-dimensional Bioprinting of Multilayered Constructs Containing Human Mesenchymal Stromal Cells for Osteochondral Tissue Regeneration in the Rabbit Knee Joint. Biofabrication, 8:014102. https://doi.org/10.1089/ten.TEA.2014.0231
56. Almarza AJ, Athanasiou KA, 2004, Seeding Techniques and Scaffolding Choice for Tissue Engineering of the Temporomandibular Joint Disk. Tissue Eng Part A, 10:1787–95. https://doi.org/10.1089/ten.2004.10.1787
57. Li L, Li J, Guo J, et al., 2019, 3D Molecularly Functionalized Cell-Free Biomimetic Scaffolds for Osteochondral Regeneration. Adv Funct Mater, 29:1807356. https://doi.org/10.1002/adfm.201807356
58. Hasani-Sadrabadi MM, Sarrion P, Nakatsuka N, et al., 2019, Hierarchically Patterned Polydopamine-Containing Membranes for Periodontal Tissue Engineering. Acs Nano, 13:3830–8. https://doi.org/10.1021/acsnano.8b09623
59. Yi WJ, Li LJ, He H, et al., 2018, Poly(L-lactide)/Cyclodextrin/Citrate Networks Modified Hydroxyapatite and its Role as Filler in the Promotion to the Properties of Poly(L-lactide) Biomaterials. Polymer, 145:1–10. https://doi.org/10.1016/j. olymer.2018.04.034
60. O’Bryan CS, Bhattacharjee T, Hart S, et al., 2017, Self-assembled Micro-Organogels for 3D Printing Silicone Structures. Sci Adv, 3:1602800. https://doi.org/10.1126/sciadv.1602800
61. Chow L, Yick KL, Kwan MY, et al., 2020, Customized Fabrication Approach for Hypertrophic Scar Treatment: 3D Printed Fabric Silicone Composite. Int J Bioprint, 6:70–81. https://doi.org/10.18063/ijb.v6i2.262
62. Luis E, Pan HM, Sing SL, et al., 2019, Silicone 3D Printing: Process Optimization, Product Biocompatibility, and Reliability of Silicone Meniscus Implants. 3D Print Addit Manufact, 6:319–32. https://doi.org/10.1089/3dp.2018.0226
63. Rahmanian M, Seyfoori A, Dehghan MM, et al., 2019, Multifunctional Gelatin-Tricalcium Phosphate Porous Nanocomposite Scaffolds for Tissue Engineering and Local Drug Delivery: In Vitro and In Vivo Studies. J Taiwan Inst Chem Eng, 101:214–20. https://doi.org/10.1016/j.jtice.2019.04.028
64. Tibbits S, 2014, 4D Printing: Multi‐Material Shape Change. Arch Des, 84:116–21. https://doi.org/10.1002/ad.1710
65. Lee JM, Yeong WY, 2016, Design and Printing Strategies in 3D Bioprinting of Cell-Hydrogels: A Review. Adv Healthc Mater, 5:2856–65. https://doi.org/10.1002/adhm.201600435
66. Vorndran E, Klammert U, Ewald A, et al., 2010, Simultaneous Immobilization of Bioactives During 3D Powder Printing of Bioceramic Drug‐Release Matrices. Adv Funct Mater, 20:1585–91. https://doi.org/10.1002/adfm.200901759
67. Chia HN, Wu BM, 2015, Recent Advances in 3D Printing of Biomaterials. J Biol Eng, 9:4. https://doi.org/10.1186/s13036-015-0001-4
68. Liu X, Ma PX, 2004, Polymeric Scaffolds for Bone Tissue Engineering. Biomaterials, 32:9622–9. https://doi.org/10.1023/b: abme.0000017544.36001.8e
69. Peltola SM, Melchels F, Grijpma DW, et al., 2008, A Review of Rapid Prototyping Techniques for Tissue Engineering Purposes. Ann Med 40:268–80. https://doi.org/10.1080/07853890701881788
70. Bajpai I, Yang S, Lee S, et al., 2015, Compressive Strength and Biomineralisation Improvement by Water Glass Coating on Porous Calcium Phosphate Scaffolds. Adv Appl Ceramics, 115:243–8. https://doi.org/10.1179/1743676115Y.0000000028
71. Shuai CJ, Yuan X, Yang WJ, et al., 2021, Synthesis of a Mace-like Cellulose [Emailprotected] Nanosystem Via In-Situ Growth for Antibacterial Activities of Poly-L-lactide scaffold. Carbohydr Polym, 262:117937. https://doi.org/10.1016/j.carbpol.2021.117937
72. Zyman ZZ, Tkachenko MV, Polevodin DV, 2008, Preparation and Characterization of Biphasic Calcium Phosphate Ceramics of Desired Composition. J Mater Sci Mater Med, 19:2819–25. https://doi.org/10.1007/s10856-008-3402-9
73. de Wild M, Amacher F, Bradbury CR, et al., 2016 Investigation of structural resorption behavior of biphasic bioceramics with help of gravimetry, μCT, SEM, and XRD. J Biomed Mater Res Part B Appl Biomater, 104:546–53. https://doi.org/10.1002/jbm.b.33419
74. Sánchez-Salcedo S, Balas F, Izquierdo-Barba I, et al., 2009, In Vitro Structural Changes in Porous HA/β-TCP Scaffolds in Simulated Body Fluid. Acta Biomater, 5:2738–51. https://doi.org/10.1016/j.actbio.2009.03.025
75. Diba M, Camargo WA, Brindisi M, et al., 2017, Composite Colloidal Gels Made of Bisphosphonate-Functionalized Gelatin and Bioactive Glass Particles for Regeneration of Osteoporotic Bone Defects. Adv Funct Mater, 27:1703438. https://doi.org/10.1002/adfm.201703438
76. Jakus AE, Rutz AL, Jordan SW, et al., 2016, Hyperelastic\bone\: A Highly Versatile, Growth Factor-free, Osteoregenerative, Scalable, and Surgically Friendly Biomaterial. Sci Transl Med, 8:358ra127. https://doi.org/10.1126/scitranslmed. aaf 7704
77. Lei M, Qu X, Liu H, et al., 2019, Programmable Electrofabrication of Porous Janus Films with Tunable Janus Balance for Anisotropic Cell Guidance and Tissue Regeneration. Adv Funct Mater, 29:1900065. https://doi.org/10.1002/adfm.201900065
78. Du Y, Liu H, Qin Y, et al., 2017, Selective Laser Sintering Scaffold with Hierarchical Architecture and Gradient Composition for Osteochondral Repair In Rabbits. Biomaterials, 137:37–48. https://doi.org/10.1016/j.biomaterials.2017.05.021.
79. Zhai X, Ruan C, Ma Y, et al., 2018, 3D-Bioprinted Osteoblast-Laden Nanocomposite Hydrogel Constructs with Induced Microenvironments Promote Cell Viability, Differentiation, and Osteogenesis both In Vitro and In Vivo. Adv Sci, 5:1700550. https://doi.org/10.1002/advs.201700550
80. Inzana JA, Olvera D, Fuller SM, et al., 2014, 3D Printing of Composite Calcium Phosphate and Collagen Scaffolds for Bone Regeneration. Biomaterials, 35:4026–34. https://doi.org/10.1016/j.biomaterials.2014.01.064
81. Li X, Zou Q, Chen H, et al., 2019, In Vivo Changes of Nanoapatite Crystals During Bone Reconstruction and the Differences with Native Bone Apatite. Sci Adv, 5:eaay6484. https://doi.org/10.1126/sciadv. aay 6484
82. Montalbano G, Molino G, Fiorilli S, et al., 2020, Synthesis and Incorporation of Rod-like Nano-hydroxyapatite into Type I Collagen Matrix: A Hybrid Formulation for 3D Printing of Bone Scaffolds. J Eur Ceramic Soc, 40:3689–97. https://doi.org/10.1016/j.jeurceramsoc.2020.02.018
83. Wang H, Li Y, Zuo Y, et al., 2007, Biocompatibility and Osteogenesis of Biomimetic Nano-hydroxyapatite/Polyamide Composite Scaffolds for Bone Tissue Engineering. Biomaterials, 28:3338–48. https://doi.org/10.1016/j.biomaterials.2007.04.014
84. Diao J, Yang JO, Deng T, et al., 2018, 3D-Plotted Beta-Tricalcium Phosphate Scaffolds with Smaller Pore Sizes Improve In Vivo Bone Regeneration and Biomechanical Properties in a Critical-Sized Calvarial Defect Rat Model. Adv Healthc Mater, 7:1800441. https://doi.org/10.1002/adhm.201800441
85. Shao H, He Y, Fu J, et al., 2016, 3D Printing Magnesium doped Wollastonite/β-TCP Bioceramics Scaffolds with High Strength and Adjustable Degradation. J Eur Ceramic Soc, 36:1495–503. https://doi.org/10.1016/j.jeurceramsoc.2016.01.010
86. Deng C, Lin R, Zhang M, et al., 2019, Micro/Nanometer-Structured Scaffolds for Regeneration of Both Cartilage and Subchondral Bone. Adv Funct Mater, 29:1806068. https://doi.org/10.1002/adfm.201806068
87. Wei Y, Gao H, Hao L, et al., 2020, Constructing a Sr2+- Substituted Surface Hydroxyapatite Hexagon-Like Microarray on 3D-Plotted Hydroxyapatite Scaffold to Regulate Osteogenic Differentiation. Nanomaterials, 10:1672. https://doi.org/10.3390/nano10091672
88. Li X, Yuan Y, Liu L, et al., 2020, 3D Printing of Hydroxyapatite/Tricalcium Phosphate Scaffold with Hierarchical Porous Structure for Bone Regeneration. Biodes Manufact, 3:15–29. https://doi.org/10.1007/s42242-019-00056-5
89. Wang C, Lai J, Li K, et al., 2020, Cryogenic 3D Printing of Dual-delivery Scaffolds for Improved Bone Regeneration with Enhanced Vascularization. Bioact Mater, 6:137–45. https://doi.org/10.1016/j.bioactmat.2020.07.007
90. Pae H, Kang J, Cha J, et al., 2019, 3D-printed Polycaprolactone Scaffold Mixed with β‐Tricalcium Phosphate as a Bone Regenerative Material in Rabbit Calvarial Defects. J Biomed Mater Res Part B Appl Biomater, 107:1254–63. https://doi.org/10.1002/jbm.b.34218
91. Shao H, Ke X, Liu A, et al., 2017, Bone Regeneration in 3D Printing Bioactive Ceramic Scaffolds with Improved Tissue/Material Interface Pore Architecture in Thin-wall Bone Defect. Biofabrication, 9:025003. https://doi.org/10.1088/1758-5090/aa663c
92. Jin ZW, Wu RH, Shen JH, et al., 2018, Nonstoichiometric Wollastonite Bioceramic Scaffolds with Core-shell Pore Struts and Adjustable Mechanical and Biodegradable Properties. Mech Behav Biomed Mater, 88:140–9. https://doi.org/10.1016/j.jmbbm.2018.08.018
93. Alksne M, Kalvaityte M, Simoliunas E, et al., 2020, In Vitro Comparison of 3D Printed Polylactic Acid/Hydroxyapatite and Polylactic Acid/Bioglass Composite Scaffolds: Insights into Materials for Bone Regeneration. J Mech Behav Biomed Mater, 104:103641. https://doi.org/10.1016/j. jmbbm.2020.103641
94. Kim WJ, Yun HS, Kim GH, 2017, An Innovative Cell-laden α-TCP/Collagen Scaffold Fabricated Using a Two-step Printing Process for Potential Application in Regenerating Hard Tissues. Sci Rep, 7:3181. https://doi.org/10.1038/s41598-017-03455-9
95. Feng C, Zhang W, Deng C, et al., 2017, 3D Printing of Lotus Root‐Like Biomimetic Materials for Cell Delivery and Tissue Regeneration. Adv Sci, 4:1700401. https://doi.org/10.1002/advs.201700401
96. Zhang M, Lin R, Wang X, et al., 2020, 3D Printing of Haversian Bone-Mimicking Scaffolds for Multicellular Delivery in Bone Regeneration. Sci Adv, 6:eaaz6725. https://doi.org/10.1126/sciadv.aaz 6725
97. Wang X, Lin M, Kang Y, 2019, Engineering Porous β-Tricalcium Phosphate (β-TCP) Scaffolds with Multiple Channels to Promote Cell Migration, Proliferation, and Angiogenesis. ACS Appl Mater Interf, 11:9223–32. https://doi.org/10.1021/acsami.8b22041
98. Chimene D, Miller L, Cross LM, et al., 2020, Nanoengineered Osteoinductive Bioink for 3D Bioprinting Bone Tissue. ACS Appl Mater Interf, 12:15976–88. https://doi.org/10.1021/acsami.9b19037
99. Moon YW, Choi IJ, Koh YH, et al., 2015, Macroporous Alumina Scaffolds Consisting of Highly Microporous Hollow Filaments Using Three-Dimensional Ceramic/Camphene-Based Co-extrusion. J Eur Ceramic Soc, 35:4623–7. https://doi.org/10.1016/j.jeurceramsoc.2015.08.017
100. Ye W, Li H, et al., 2020, 3D Printing of Gelatin Methacrylate based Nerve Guidance Conduits with Multiple Channels. Mater Des, 192:108757. https://doi.org/10.1016/j.matdes.2020.108757
101. Ke X, Zhuang C, Yang X, et al., 2017, Enhancing the Osteogenic Capability of Core-Shell Bilayered Bioceramic Microspheres with Adjustable Biodegradation. ACS Appl Mater Interf, 9:24497–510. https://doi.org/10.1021/acsami.7b06798
102. Pistry P, Aied A, Alexander M, et al., 2017, Bioprinting Using Mechanically Robust Core–Shell Cell‐Laden Hydrogel Strands. Macromol Biosci, 17:1600472. https://doi.org/10.1002/mabi.201600472
103. Taymour R, Kilian D, Ahlfeld T, et al., 2021, 3D Bioprinting of Hepatocytes: Core-shell Structured Co-cultures with Fibroblasts for Enhanced Functionality. Sci Rep, 11:5130. https://doi.org/10.1038/s41598-021-84384-6
104. Hong SY, Ji SK, Jung B, et al., 2019, Coaxial Bioprinting of Cell-laden Vascular Constructs Using a Gelatin-tyramine Bioink. Biomater Sci, 7:4578–87. https://doi.org/10.1039/c8bm00618k
105. Cui J, Wang H, Shi Q, et al., 2019, Multicellular Co-Culture in Three-Dimensional Gelatin Methacryloyl Hydrogels for Liver Tissue Engineering. Molecules, 24:1762. https://doi.org/10.3390/molecules24091762
106. Han X, Sun M, Chen B, et al., 2021, Lotus Seedpod-inspired Internal Vascularized 3D Printed Scaffold for Bone Tissue Repair. Bioact Mater, 6:1639–52. https://doi.org/10.1016/j.bioactmat.2020.11.019
107. Gao Q, Liu Z, Lin Z, et al., 2017, 3D Bioprinting of Vessel like Structures with Multi-level Fluidic Channels. ACS Biomater Sci Eng, 3:399–408. https://doi.org/10.1021/acsbiomaterials.6b00643
108. Ma X, Xin Q, Wei Z, et al., 2016, Deterministically Patterned Biomimetic Human iPSC-Derived Hepatic Model Via Rapid 3D Bioprinting. Proc Natl Acad Sci, 113:2206. https://doi.org/10.1073/pnas.1524510113
109. Xie M, Yu K, Yuan K, et al., 2019, Protocols of 3D Bioprinting of Gelatin Methacryloyl Hydrogel Based Bioinks. J Vis Exp, 154:e60545. https://doi.org/10.3791/60545
110. Xue JM, Feng C, Xia LG, et al., 2018, Assembly Preparation of Multi layered Biomaterials with High Mechanical Strength and Bone-Forming Bioactivity. Chem Mater, 30:4646–57. https://doi.org/10.1021/acs.chemmater.8b01272
111. Mitsouras D, Liacouras P, Imanzadeh A, et al., 2015, Medical 3D Printing for the Radiologist. Radiographics, 35:1965–88. https://doi.org/10.1148/rg.2015140320
112. Hallem A, Javaid M, Saxena A, 2018, Additive Manufacturing Applications in Cardiology: A Review. Egypt Heart J, 70:433–41. https://doi.org/10.1016/j.ehj.2018.09.008
113. Odeh M, Levin D, Inziello J, et al., 2019, Methods for Verification of 3D Printed Anatomic Model Accuracy Using Cardiac Models as an Example. 3D Print Med, 5:6. https://doi.org/10.1186/s41205-019-0043-1
114. Vukicevic M, Mosadegh B, Min JK, et al., 2017, Cardiac 3D Printing and its Future Directions. Jacc Cardiovasc Imaging, 10:171–84. https://doi.org/10.1016/j.jcmg.2016.12.001
115. Doucet G, Ryan S, Bartellas M, et al., 2017, Modelling and Manufacturing of a 3D Printed Trachea for Cricothyroidotomy Simulation. Cureus, 9:e1575. https://doi.org/10.7759/cureus.1575
116. Jin ZW, Li Y, Yu K, et al., 2021, 3D Printing of Physical Organ Models: Recent Developments and Challenges. Adv Sci, 2021:2101394. https://doi.org/10.1002/advs.202101394
117. Hollister SJ. Hollister SJ, 2005, Porous Scaffold Design for Tissue Engineering. Nat Mater, 4:518–24. https://doi.org/10.1038/nmat1421
118. Hutmacher DW, 2000, Scaffolds in Tissue Engineering Bone and Cartilage. Biomaterials, 21:2529–43. https://doi.org/10.1016/S0142-9612(00)00121-6
119. Vaz CM, Tuijl S V, Bouten C, et al., 2005, Design of Scaffolds for Blood Vessel Tissue Engineering Using a Multi-layering Electrospinning Technique. Acta Biomater, 1:575–82. https://doi.org/10.1016/j.actbio.2005.06.006
120. Ma PX, 2004, Scaffolds for Tissue Fabrication. Mater Today, 7:30–40. https://doi.org/10.1016/S1369-7021(04)00233-0
121. Zhang YS, Yue K, Aleman J, et al., 2017, 3D Bioprinting for Tissue and Organ Fabrication. Ann Biomed Eng, 45:148–63. https://doi.org/10.1007/s10439-016-1612-8
122. Yeo MG, Lee JS, Chun W, et al., 2016, An Innovative Collagen-Based Cell-Printing Method for Obtaining Human Adipose Stem Cell-Laden Structures Consisting of Core-Sheath Structures for Tissue Engineering. Biomacromolecules, 17:1365–75. https://doi.org/10.1021/acs.biomac.5b01764
123. Yeo MG, Ha JH, Lee HJ, et al., 2016, Fabrication of hASCsladen Structures Using Extrusion-based Cell Printing Supplemented with an Electric Field. Acta Biomater, 38:33–43. https://doi.org/10.1016/j.actbio.2016.04.017
124. Xu T, Gregory CA, Molnar P, et al., 2006, Viability and Electrophysiology of Neural Cell Structures Generated by the Inkjet Printing Method. Biomaterials, 27:3580–8. https://doi.org/10.1016/j.biomaterials.2006.01.048
125. Xu F, Finley TD, Turkaydin M, et al., 2011, The Assembly of Cell-encapsulating Microscale Hydrogels Using Acoustic Waves. Biomaterials, 32:7847–55. https://doi.org/10.1016/j.biomaterials.2011.07.010
126. Koo Y W, Kim GH, 2016, New Strategy for Enhancing In Situ Cell Viability of Cell-printing Process Via Piezoelectric Transducer-assisted Three-Dimensional Printing. Biofabrication, 8:025010. https://doi.org/10.1088/1758-5090/8/2/025010
127. Koch L, Deiwick A, Chichkov B, 2014, Laser-based 3D Cell Printing for Tissue Engineering. Bionanomaterials, 15:71–8. https://doi.org/10.1515/bnm-2014-0005
128. Furukawa KS, Imura K, Tateishi T, et al., 2008, Scaffold free Cartilage by Rotational Culture for Tissue Engineering. J Biotechnol, 133:134–45. https://doi.org/10.1016/j.jbiotec.2007.07.957
129. Keeney M, Pandit A, 2009, The Osteochondral Junction and its Repair via Bi-Phasic Tissue Engineering Scaffolds. Tissue Eng Part B Rev, 15:55–73. https://doi.org/10.1089/ten.teb.2008.0388
130. Zhang H, Huang H, Hao G, et al., 2020, 3D Printing Hydrogel Scaffolds with Nanohydroxyapatite Gradient to Effectively Repair Osteochondral Defects in Rats. Adv Funct Mater, 31:2006697. https://doi.org/10.1002/adfm.202006697
131. Amini AR, Laurencin CT, Nukavarapu SP, 2012, Bone Tissue Engineering: Recent Advances and Challenges. Crit Rev Biomed Eng, 40:363–408. https://doi.org/10.1615/critrevbiomedeng. v40. i5.10
132. Rezwan K, Chen QZ, Blaker JJ, et al., 2006, Biodegradable and Bioactive Porous Polymer/Inorganic Composite Scaffolds for Bone Tissue Engineering. Biomaterials, 27:3413–31. https://doi.org/10.1016/j. biomaterials.2006.01.039
133. Asadi N, Alizadeh E, Salehi R, et al., 2017, Nanocomposite Hydrogels for Cartilage Tissue Engineering: A Review. Artif Cells Nanomed Biotechnol, 46:465–71. https://doi.org/10.1080/21691401.2017.1345924
134. Chen J, Yang J, Wang L, et al., 2021, Modified Hyaluronic Acid Hydrogels with Chemical Groups that Facilitate Adhesion to Host Tissues Enhance Cartilage Regeneration. Bioact Mater, 6:1689–98. https://doi.org/10.1016/j.bioactmat.2020.11.020
135. Sun Y, You Y, Jiang W, et al., 2020, 3D Bioprinting Dual factor Releasing and Gradient-structured Constructs Ready to Implant for Anisotropic Cartilage Regeneration. Sci Adv, 6:eaay1422. https://doi.org/10.1126/sciadv. aay 1422
136. Diloksumpan P, Castilho M, Gbureck U, et al., 2020, Combining Multi-Scale 3D Printing Technologies to Engineer Reinforced Hydrogel-ceramic Interfaces. Biofabrication, 12:025014. https://doi.org/10.1088/1758-5090/ab69d9
137. Kim SH, Yeon YK, Lee JM, et al., 2018, Precisely Printable and Biocompatible Silk Fibroin Bioink for Digital Light Processing 3D Printing. Nat Commun, 9:1620. https://doi.org/10.1038/s41467-018-03759-y
138. Hong H, Seo YB, Kim DY, et al., 2020, Digital Light Processing 3D Printed Silk Fibroin Hydrogel for Cartilage Tissue Engineering. Biomaterials, 232:119679. https://doi.org/10.1016/j.biomaterials.2019.119679
139. Bae H, Puranik A, Gauvin R, et al., 2012, Building Vascular Networks. Sci Transl Med, 4:160. https://doi.org/10.1126/scitranslmed.3003688
140. Nomi M, Atala A, Coppi PD, et al., 2003, Principals of Neovascularization for Tissue Engineering. Mol Aspect Med, 23:463–83. https://doi.org/10.1016/s0098-2997(02)00008-0
141. Novosel EC, Kleinhans C, Kluger PJ, 2011, Vascularization is the Key Challenge in Tissue Engineering. Adv Drug Deliv Rev, 63:300–11. https://doi.org/10.1016/j.addr.2011.03.004
142. Zhang YS, Khademhosseini A, 2015, Seeking the Right Context for Evaluating Nanomedicine: From Tissue Models in Petri Dishes to Microfluidic Organs-on-a-Chip. Nanomedicine, 10:685–8. https://doi.org/10.2217/nnm.15.18
143. Jia W, Gungor-Ozkerim PS, Yu SZ, et al., 2016, Direct 3D Bioprinting of Perfusable Vascular Constructs Using a Blend Bioink. Biomaterials, 106:58–68. https://doi.org/10.1016/j.biomaterials.2016.07.038
144. Suntornnond R, Tan E, An J, et al., 2017, A Highly Printable and Biocompatible Hydrogel Composite for Direct Printing of Soft and Perfusable Vasculature-like Structures. Sci Rep, 7:16902. https://doi.org/10.1038/s41598-017-17198-0
145. Zhang Y, Yu Y, Chen H, et al., 2013, Characterization of Printable Cellular Micro-fluidic Channels for Tissue Engineering. Biofabrication, 5:025004. https://doi.org/10.1088/1758-5082/5/2/025004
146. Isakoff MS, Bielack SS, Meltzer P, et al., 2015, Osteosarcoma: Current Treatment and a Collaborative Pathway to Success. J Clin Oncol, 33:3029–35. https://doi.org/10.1200/JCO.2014.59.4895
147. Italiano A, Mir O, Cioffi A, et al., 2013, Advanced Chondrosarcomas: Role of Chemotherapy and Survival. Ann Oncol, 24:2916–22. https://doi.org/10.1093/annonc/mdt374
148. Gaspar N, Hawkins DS, Dirksen U, et al., 2016, Ewing Sarcoma: Current Management and Future Approaches Through Collaboration. J Clin Oncol, 33:3036–46. https://doi.org/10.1200/JCO.2014.59.5256
149. Smeland S, Bielack SS, Whelan J, et al., 2019, Survival and Prognosis with Osteosarcoma: Outcomes in more than 2000 Patients in the EURAMOS-1 (European and American Osteosarcoma Study) Cohort. Eur J Cancer, 109:36–50. https://doi.org/10.1016/j.ejca.2018.11.027
150. Hu MC, Yao ZH, Liu XG, et al., 2018, Enhancement Mechanism of Hydroxyapatite for Photocatalytic Degradation of Gaseous Formaldehyde over TiO2/Hydroxyapatite. J Taiwan Inst Chem Eng, 85:91–7. https://doi.org/10.1016/j.jtice.2017.12.021
151. Kebede MA, Asiku KS, Imae T, et al., 2018, Stereolithographic and Molding Fabrications of Hydroxyapatite-polymer Gels Applicable to Bone Regeneration Materials. J Taiwan Inst Chem Eng, 92:91–6. https://doi.org/10.1016/j.jtice.2018.01.034
152. Huang L, Lu W, Liu M, et al., 2017, Facile Preparation of Eu3+ and F co-Doped Luminescent Hydroxyapatite Polymer Composites via the Photo-RAFT Polymerization. J Taiwan Inst Chem Eng, 83:184–91. https://doi.org/10.1016/j.jtice.2017.12.006
153. Mehdi R, Amir S, Mohammad MD, et al., 2019, Multifunctional Gelatin Tricalcium Phosphate Porous Nanocomposite Scaffolds for Tissue Engineering and Local Drug Delivery: In Vitro and In Vivo Studies. J Taiwan Inst Chem Eng, 101:214–20. https://doi.org/10.1016/j. jtice.2019.04.028
154. Cheng L, Wang C, Feng L, et al., 2014, Functional Nanomaterials for Phototherapies of Cancer. Chin J Clin Oncol, 114:10869–939. https://doi.org/10.1021/cr400532z
155. Ma H, Jiang C, Dong Z, et al., 2016, A Bifunctional Biomaterial with Photothermal Effect forTumor Therapy and Bone Regeneration. Adv Funct Mater, 26:1197–208. https://doi.org/10.1021/cr400532z
156. Qu Y, Chu BY, Peng JR, et al., 2015, A Biodegradable Thermo responsive Hybrid Hydrogel: Therapeutic Applications in Preventing the Post-operative Recurrence of Breast Cancer. Npg Asia Mater, 7:e207. https://doi.org/10.1038/am.2015.83
157. Wang X, Li T, Ma H, et al., 2017, A 3D-printed Scaffold with MoS2 Nanosheets for Tumor Therapy and Tissue Regeneration. Npg Asia Mater, 9:e376. https://doi.org/10.1038/am.2017.47
158. Liu HH, Lin ML, Liu X, et al., 2020, Doping Bioactive Elements into a Collagen Scaffold Based on Synchronous Self-assembly/Mineralization for Bone Tissue Engineering. Bioactive Mater, 5:844–58. https://doi.org/10.1016/j. bioactmat.2020.06.005
159. Wang XC, Xue JM, Ma B, et al., 2020, Black Bioceramics: Combining Regeneration with Therapy. Adv Mater, 32:e2005140. https://doi.org/10.1002/adma.202005140
160. Bo L, Wang X, Lei C, et al., 2018, Ultrathin Cu-TCPP MOF Nanosheets: A New Theragnostic Nanoplatform with Magnetic Resonance/Near-infrared Thermal Imaging for Synergistic Phototherapy of Cancers. Theranostics, 8:4086–96. https://doi.org/10.7150/thno.25433