3D Printing Polymer-based Bolus Used for Radiotherapy
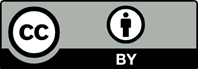
Bolus is a kind of auxiliary device used in radiotherapy for the treatment of superficial lesions such as skin cancer. It is commonly used to increase skin dose and overcome the skin-sparing effect. Despite the availability of various commercial boluses, there is currently no bolus that can form full contact with irregular surface of patients’ skin, and incomplete contact would result in air gaps. The resulting air gaps can reduce the surface radiation dose, leading to a discrepancy between the delivered dose and planned dose. To avoid this limitation, the customized bolus processed by three-dimensional (3D) printing holds tremendous potential for making radiotherapy more efficient than ever before. This review mainly summarized the recent development of polymers used for processing bolus, 3D printing technologies suitable for polymers, and customization of 3D printing bolus. An ideal material for customizing bolus should not only have the feature of 3D printability for customization, but also possess radiotherapy adjuvant performance as well as other multiple compound properties, including tissue equivalence, biocompatibility, antibacterial activity, and antiphlogosis.
1. Baskar R, Lee KA, Yeo R, et al., 2012, Cancer and Radiation Therapy: Current Advances and Future Directions. Int J Med Sci, 9:193–9.
2. Hodapp N, 2012, The ICRU Report 83: Prescribing, Recording and Reporting Photon-Beam Intensity-Modulated Radiation Therapy (IMRT). Strahlenther Onkol, 188:97–9. https://doi.org/10.1093/jicru_ndq002
3. Babic S, Kerr A T, Westerland M, et al., 2002, Examination of Jeltrate Plus as a Tissue Equivalent Bolus Material. J Appl Clin Med Phys, 3:170–5. https://doi.org/10.1120/1.1471552
4. Hsu SH, Roberson PL, Chen Y, et al., 2008, Assessment of Skin Dose for Breast Chest Wall Radiotherapy as a Function of Bolus Material. Phys Med Biol, 53:2593–606. https://doi.org/10.1088/0031-9155/53/10/010
5. Aras S, Tanzer İO, 2020, Dosimetric comparison of superflab and specially prepared bolus materials used in radiotherapy practice. Eur J Breast Health, 16:167–70. https://doi.org/10.5152/ejbh.2020.5041
6. Khan Y, Villarreal-Barajas JE, Udowicz M, et al., 2013, Clinical and Dosimetric Implications of Air Gaps between Bolus and Skin Surface during Radiation Therapy. J Cancer Ther, 4:1251–5. https://doi.org/10.4236/jct.2013.47147
7. Hou Y, Song Y, Sun X, et al., 2020, Multifunctional Composite Hydrogel Bolus with Combined Self-Healing, Antibacterial and Adhesive Functions for Radiotherapy. J Mater Chem B, 8:2627–35. https://doi.org/10.1039/c9tb02967b
8. Dipasquale G, Poirier A, Sprunger Y, et al., 2018, Improving 3D-Printing of Megavoltage X-Rays Radiotherapy Bolus with Surface-Scanner. Radiat Oncol, 13:203. https://doi.org/10.1186/s13014-018-1148-1
9. Baltz GC, Chi PM, Wong PF, et al., 2019, Development and Validation of a 3D-Printed Bolus Cap for Total Scalp Irradiation. J Appl Clin Med Phys, 20:89–96. https://doi.org/10.1002/acm2.12552
10. Kong Y, Yan T, Sun Y, et al., 2019, A Dosimetric Study on the Use of 3D-Printed Customized Boluses in Photon Therapy: A Hydrogel and Silica Gel Study. J Appl Clin Med Phys, 20:348–55. https://doi.org/10.1002/acm2.12489
11. Robar JL, Moran K, Allan J, et al., 2018, Intrapatient Study Comparing 3D Printed Bolus Versus Standard Vinyl Gel Sheet Bolus for Postmastectomy Chest Wall Radiation Therapy. Pract Radiat Oncol, 8:221–9. https://doi.org/10.1016/j.prro.2017.12.008
12. Aoyama T, Uto K, Shimizu H, et al., 2020, Physical and Dosimetric Characterization of Thermoset Shape Memory Bolus Developed for Radiotherapy. Med Phys, 47:6103–12. https://doi.org/10.1002/mp.14516
13. Asfia A, Novak JI, Mohammed MI, et al., 2020, A Review of 3D Printed Patient Specific Immobilisation Devices in Radiotherapy. Phys Imaging Radiat Oncol, 13:30–5. https://doi.org/10.1016/j.phro.2020.03.003
14. Zemnick C, Woodhouse SA, Gewanter RM, et al., 2007, Rapid Prototyping Technique for Creating a Radiation Shield. J Prosthet Dent, 97:236–41. https://doi.org/10.1016/j.prosdent.2007.02.005
15. Zhao Y, Moran K, Yewondwossen M, et al., 2017, Clinical Applications of 3-Dimensional Printing in Radiation Therapy. Med Dosim, 42:150–5.
16. Tino R, Leary M, Yeo A, et al., 2020, Additive Manufacturing in Radiation Oncology: A Review of Clinical Practice, Emerging Trends and Research Opportunities. Int J Extrem Manuf, 2:012003. https://doi.org/10.1088/2631-7990/ab70af
17. Walker M, Cohen N, Menchaca D, 2005, Play-Doh and Water-Soaked Gauze Sponges as Alternative Bolus Material For Cobalt-60 Teletherapy. Vet Radiol Ultrasound, 46:179–81. https://doi.org/10.1111/j.1740-8261.2005.00033.x
18. Vyas V, Palmer L, Mudge R, et al., 2013, On Bolus for Megavoltage Photon and Electron Radiation Therapy. Med Dosim, 38:268–73. https://doi.org/10.1016/j.meddos.2013.02.007
19. Rus D, Tolley MT, 2015, Design, Fabrication and Control of soft Robots. Nature, 521:467–75. https://doi.org/10.1038/nature14543
20. Benoit J, Pruitt AF, Thrall DE, 2009, Effect of Wetness Level on the Suitability of Wet Gauze as a Substitute for Superflab as a Bolus Material for use with 6mv Photons. Vet Radiol Ultrasound, 50:555–9. https://doi.org/10.1111/j.1740-8261.2009.01573.x
21. Reft CS, 1989, Output Calibration in Solid Water for High Energy Photon Beams. Med Phys, 16:299–301. https://doi.org/10.1118/1.596423
22. Kim SW, Shin HJ, Kay CS, et al., 2014, A customized Bolus Produced Using a 3-Dimensional Printer for Radiotherapy. PLoS One, 9:e110746. https://doi.org/10.1371/journal.pone.0110746
23. Lukowiak M, Jezierska K, Boehlke M, et al., 2017, Utilization of a 3D Printer to Fabricate Boluses used for Electron Therapy of Skin Lesions of the Eye Canthi. J Appl Clin Med Phys, 18:76–81.
24. Park SY, Choi CH, Park JM, et al., 2016, A Patient-Specific Polylactic Acid Bolus Made by a 3D Printer for Breast Cancer Radiation Therapy. PLoS One, 11:e0168063. https://doi.org/10.1371/journal.pone.0168063
25. Banerjee SL, Samanta S, Sarkar S, et al., 2020, A Self-Healable and Antifouling Hydrogel Based on PDMS Centered ABA Tri-Block Copolymer Polymersomes: A Potential Material for Therapeutic Contact Lenses. J Mater Chem B, 8:226–43. https://doi.org/10.1039/c9tb00949c
26. Chiu T, Tan J, Brenner M, et al., 2018, Three-Dimensional Printer-Aided Casting of Soft, Custom Silicone Boluses (SCSBs) for Head and Neck Radiation Therapy. Pract Radiat Oncol, 8:e167–4. https://doi.org/10.1016/j.prro.2017.11.001
27. Chiozzini GC, Mendes GP, Vanni FP, et al., 2020, Bacterial nanocellulose membrane as bolus in radiotherapy: “Proof of concept”. Cellulose, 28:607–13. https://doi.org/10.1007/s10570-020-03579-8
28. Cooke SL, Whittington AR, 2016, Influence of Therapeutic Radiation on Polycaprolactone and Polyurethane Biomaterials. Mater Sci Eng C Mater Biol Appl, 60:78–83.
29. Bil M, Kijeńska-Gawrońska E, Głodkowska-Mrówka E, et al., 2020, Design and In Vitro Evaluation of Electrospun Shape Memory Polyurethanes for Self-Fitting Tissue Engineering Grafts and Drug Delivery Systems. Mater Sci Eng C Mater Biol Appl, 110:110675. https://doi.org/10.1016/j.msec.2020.110675
30. Eslahi N, Abdorahim M, Simchi A, 2016, Smart Polymeric Hydrogels for Cartilage Tissue Engineering: A Review on the Chemistry and Biological Functions. Biomacromolecules, 17:3441–63. https://doi.org/10.1021/acs.biomac.6b01235
31. Ng WL, Chua CK, Shen YF, 2019, Print Me An Organ! Why We Are Not There Yet. Prog Polym Sci, 97:101145. https://doi.org/10.1016/j.progpolymsci.2019.101145
32. Askari M, Naniz MA, Kouhi M, et al., 2021, Recent Progress in Extrusion 3D Bioprinting of Hydrogel Biomaterials for Tissue Regeneration: A Comprehensive Review with Focus on Advanced Fabrication Techniques. Biomater Sci, 9:535–73. https://doi.org/10.1039/d0bm00973c
33. Tao G, Wang Y, Cai R, et al., 2019, Design and Performance of Sericin/Poly(Vinyl Alcohol) Hydrogel as a Drug Delivery Carrier for Potential Wound Dressing Application. Mater Sci Eng C Mater Biol Appl, 101:341–51. https://doi.org/10.1016/j.msec.2019.03.111
34. Wang T, Qu G, Wang C, et al., 2019, Importance of Polyacrylamide Hydrogel Diverse Chains and Cross-Linking Density for Cell Proliferation, Aging, and Death. Langmuir, 35:13999–4006. https://doi.org/10.1021/acs.langmuir.9b02799.s001
35. An H, Zhu L, Shen J, et al., 2020, Self-Healing PEG-Poly (Aspartic Acid) Hydrogel with Rapid Shape Recovery and Drug Release. Colloids Surf B Biointerfaces, 185:110601. https://doi.org/10.1016/j.colsurfb.2019.110601
36. Hussain Z, Thu HE, Shuid AN, et al., 2018, Recent Advances in Polymer-based Wound Dressings for the Treatment of Diabetic Foot Ulcer: An Overview of State-of-the-Art. Curr Drug Targets, 19:527–50. https://doi.org/10.2174/1389450118666170704132523
37. Bonani W, Cagol N, Maniglio D, 2020, Alginate Hydrogels: A Tool for 3D Cell Encapsulation, Tissue Engineering, and Biofabrication. Adv Exp Med Biol, 1250:49–61. https://doi.org/10.1007/978-981-15-3262-7_4
38. Xu J, Zheng S, Hu X, 2020, Advances in the Research of Bioinks Based on Natural Collagen, Polysaccharide and Their Derivatives for Skin 3D Bioprinting. Polymers (Basel), 12:1237. https://doi.org/10.3390/polym12061237
39. Haq MA, Su Y, Wang D, 2017, Mechanical Properties of PNIPAM Based Hydrogels: A Review. Mater Sci Eng C Mater Biol Appl, 70:842–55.
40. Zhang B, Li S, Hingorani H, et al., 2018, Highly Stretchable Hydrogels for UV Curing Based High-Resolution Multimaterial 3D Printing. J Mater Chem B, 6:3246–53. https://doi.org/10.1039/c8tb00673c
41. Sun JY, Zhao X, Illeperuma WR, et al., 2012, Highly Stretchable and Tough Hydrogels. Nature, 489:133–6.
42. Truby RL, Lewis JA, 2016, Printing Soft Matter in Three Dimensions. Nature, 540:371–8. https://doi.org/10.1038/nature21003
43. Zhu W, Ma X, Gou M, et al., 2016, 3D Printing of Functional Biomaterials for Tissue Engineering. Curr Opin Biotechnol, 40:103–12.
44. Murphy SV, Atala A, 2014, 3D Bioprinting of Tissues and Organs. Nat Biotechnol, 32:773–85. https://doi.org/10.1038/nbt.2958
45. Zhou LY, Fu J, He Y, 2020, A Review of 3D Printing Technologies for Soft Polymer Materials. Adv Funct Mater, 30:2000187. https://doi.org/10.1002/adfm.202000187
46. Kinstlinger IS, Bastian A, Paulsen SJ, et al., 2016, Open-Source Selective Laser Sintering (OpenSLS) of Nylon and Biocompatible Polycaprolactone. PLoS One, 11:e0147399. https://doi.org/10.1371/journal.pone.0147399
47. Soe SP, Eyers DR, Setchi R, 2013, Assessment of Non-Uniform Shrinkage in the Laser Sintering of Polymer Materials. Int J Adv Manuf Technol, 68:111–25. https://doi.org/10.1007/s00170-012-4712-0
48. Liu K, Sun H, Tan Y, et al., 2016, Additive Manufacturing of Traditional Ceramic Powder Via Selective Laser Sintering with Cold Isostatic Pressing. Int J Adv Manuf Technol, 90:945–52. https://doi.org/10.1007/s00170-016-9441-3
49. Novitsky TF, Mathias LJ, Osborn S, et al., 2012, Synthesis and Thermal Behavior of Polyamide 12, T Random and Block Copolymers. Macromol Symp, 313–314:90–9. https://doi.org/10.1002/masy.201250310
50. Gu D, Wang H, Zhang G, 2014, Selective Laser Melting Additive Manufacturing of Ti-Based Nanocomposites: The Role of Nanopowder. Metall Mater Trans A, 45:464–76. https://doi.org/10.1007/s11661-013-1968-4
51. Ramanath HS, Chua CK, Leong KF, et al., 2008, Melt Flow Behaviour of Poly-Epsilon-Caprolactone in Fused Deposition Modelling. J Mater Sci Mater Med, 19:2541–50. https://doi.org/10.1007/s10856-007-3203-6
52. Heras ES, Haro FB, 2018, Filament Advance Detection Sensor for Fused Deposition Modelling 3D Printers. Sensors (Basel), 18:1495. https://doi.org/10.3390/s18051495
53. Hrynevich A, Elçi B, Haigh JN, et al., 2018, Dimension-Based Design of Melt Electrowritten Scaffolds. Small, 14:e1800232. https://doi.org/10.1002/smll.201800232
54. Cheng Y, Chan KH, Wang XQ, et al., 2019, Direct-Ink-Write 3D Printing of Hydrogels into Biomimetic Soft Robots. ACS Nano, 13:13176–84. https://doi.org/10.1021/acsnano.9b06144
55. Paxton N, Smolan W, Böck T, et al., 2017, Proposal to Assess Printability of Bioinks for Extrusion-Based Bioprinting and Evaluation of Rheological Properties Governing Bioprintability. Biofabrication, 9:044107. https://doi.org/10.1088/1758-5090/aa8dd8
56. Zhou LY, 2019, Multimaterial 3D Printing of Highly Stretchable Silicone Elastomers. ACS Appl Mater Interfaces, 11:23573–83. https://doi.org/10.1021/acsami.9b04873
57. Kim Y, Yuk H, Zhao R, et al., 2018, Printing Ferromagnetic Domains for Untethered Fast-Transforming Soft Materials. Nature, 558:274–9. https://doi.org/10.1038/s41586-018-0185-0
58. Kuang M, Wang L, Song Y, 2014, Controllable Printing Droplets for High-Resolution Patterns. Adv Mater, 26:6950–8. https://doi.org/10.1002/adma.201305416
59. Scoutaris N, Ross S, Douroumis D, 2016, Current Trends on Medical and Pharmaceutical Applications of Inkjet Printing Technology. Pharm Res, 33:1799–816. https://doi.org/10.1007/s11095-016-1931-3
60. Negro A, Cherbuin Tm Lutolf MP, 2018, 3D Inkjet Printing of Complex, Cell-Laden Hydrogel Structures. Sci Rep, 8:17099. https://doi.org/10.1038/s41598-018-35504-2
61. Ehtezazi T, Dempster NM, Martin GD, et al., 2014, Development of High-Throughput Glass Inkjet Devices for Pharmaceutical Applications. J Pharm Sci, 103:3733–42. https://doi.org/10.1002/jps.24192
62. Poozesh S, Saito K, Akafuah NK, et al., 2016, Comprehensive Examination of a New Mechanism to Produce Small Droplets in Drop-on-Demand Inkjet Technology. Appl Phys A, 122:110. https://doi.org/10.1007/s00339-016-9630-9
63. Li W, Mille LS, Robledo JA, et al., 2020, Recent Advances in Formulating and Processing Biomaterial Inks for Vat Polymerization‐Based 3D Printing. Adv Healthc Mater, 9:2000156. https://doi.org/10.1002/adhm.202000156
64. Ng WL, Lee JM, Zhou M, et al., 2020, Vat Polymerization-Based Bioprinting-Process, Materials, Applications and Regulatory Challenges. Biofabrication, 12:022001. https://doi.org/10.1088/1758-5090/ab6034
65. Melchels FP, Feijen J, Grijpma DW, 2010, A Review on Stereolithography and its Applications in Biomedical Engineering. Biomaterials, 31:6121–30. https://doi.org/10.1016/j.biomaterials.2010.04.050
66. Kumar H, Kim K, 2020, Stereolithography 3D Bioprinting. Methods Mol Biol, 2140:93–108.
67. Laza SC, Polo M, Neves AA, et al., 2012, Two-Photon Continuous Flow Lithography. Adv Mater, 24:1304–8. https://doi.org/10.1002/adma.201103357
68. Annabi N, Tamayol A, Uquillas JA, et al., 2014, 25th Anniversary Article: Rational Design and Applications of Hydrogels in Regenerative Medicine. Adv Mater, 26:85–123. https://doi.org/10.1002/adma.201303233
69. Ho CM, Mishra A, Hu K, et al., 2017, Femtosecond-Laser-Based 3D Printing for Tissue Engineering and Cell Biology Applications. ACS Biomater Sci Eng, 3:2198–214. https://doi.org/10.1021/acsbiomaterials.7b00438
70. Gauvin R, Chen YC, Lee JW, et al., 2012, Microfabrication of Complex Porous Tissue Engineering Scaffolds using 3D Projection Stereolithography. Biomaterials, 33:3824–34. https://doi.org/10.1016/j.biomaterials.2012.01.048
71. Kelly BE, Bhattacharya I, 2019, Volumetric Additive Manufacturing Via Tomographic Reconstruction. Science, 363:1075–9.
72. Raman R, Bhaduri B, Mir M, et al., 2016, High-Resolution Projection Microstereolithography for Patterning of Neovasculature. Adv Healthc Mater, 5:610–9. https://doi.org/10.1002/adhm.201500721
73. Xu X, Awad A, Robles-Martinez P, et al., 2021, Vat Photopolymerization 3D Printing for Advanced Drug Delivery and Medical Device Applications. J Control Release, 329:743–57. https://doi.org/10.1016/j.jconrel.2020.10.008
74. Mostafa KG, Arshad M, Ullah A, et al., 2020, Concurrent Modelling and Experimental Investigation of Material Properties and Geometries Produced by Projection Microstereolithography. Polymers (Basel), 12:506. https://doi.org/10.3390/polym12030506
75. Ligon SC, Liska R, 2017, Polymers for 3D Printing and Customized Additive Manufacturing. Chem Rev, 117:10212–90. https://doi.org/10.1021/acs.chemrev.7b00074
76. Park JW, Oh SA, Yea JW, et al., 2017, Fabrication of Malleable Three-Dimensional-Printed Customized Bolus Using Three-Dimensional Scanner. PLoS One, 12:e0177562. https://doi.org/10.1371/journal.pone.0177562
77. Kang D, Wang B, Peng Y, et al., 2020, Low-Cost iPhone-Assisted Processing to Obtain Radiotherapy Bolus Using Optical Surface Reconstruction and 3D-Printing. Sci Rep, 10:8016. https://doi.org/10.1038/s41598-020-64967-5
78. Robertson FM, Couper MB, Kinniburgh M, et al., 2021, Ninjaflex vs Superflab: A Comparison of Dosimetric Properties, Conformity to the Skin Surface, Planning Target Volume Coverage and Positional Reproducibility for External Beam Radiotherapy. J Appl Clin Med Phys, 22:26–33. https://doi.org/10.1002/acm2.13147
79. An HJ, Kim MS, Kim J, et al., 2019, Geometric Evaluation of Patient-Specific 3D Bolus from 3D Printed Mold and Casting Method for Radiation Therapy. Prog Med Phys, 30:32. https://doi.org/10.14316/pmp.2019.30.1.32
80. Burleson S, Baker J, Hsia AT, et al., 2015, Use of 3D Printers to Create a Patient-Specific 3D Bolus for External Beam Therapy. J Appl Clin Med Phys, 16:5247. https://doi.org/10.1118/1.4889472
81. Park JM, Son J, An HJ, et al., 2019, Bio-Compatible Patient-Specific Elastic Bolus for Clinical Implementation. Phys Med Biol, 64:105006. https://doi.org/10.1088/1361-6560/ab1c93
82. Park JW, Yea JW, 2016, Three-Dimensional Customized Bolus for Intensity-Modulated Radiotherapy in a Patient with Kimura’s Disease Involving the Auricle. Cancer Radiother, 20:205–9. https://doi.org/10.1016/j.canrad.2015.11.003
83. Obeid JP, Gutkin PM, Lewis J, et al., 2019, Volumetric Modulated Arc Therapy and 3-Dimensional Printed Bolus in the Treatment of Refractory Primary Cutaneous Gamma Delta Lymphoma of the Bilateral Legs. Pract Radiat Oncol, 9:220–5. https://doi.org/10.1016/j.prro.2019.02.016
84. Munoz L, Rijken J, Hunter M, et al., 2020, Investigation of Elastomeric Materials for Bolus using Stereolithography Printing Technology in Radiotherapy. Biomed Phys Eng Express, 6:045014. https://doi.org/10.1088/2057-1976/ab9425
85. Tack P, Victor J, Gemmel P, et al., 2016, 3D-Printing Techniques in a Medical Setting: A Systematic Literature Review. Biomed Eng Online, 15:115. https://doi.org/10.1186/s12938-016-0236-4
86. Thrasher CJ, Schwartz JJ, Boydston AJ, 2017, Modular Elastomer Photoresins for Digital Light Processing Additive Manufacturing. ACS Appl Mater Interfaces, 9:39708– .https://doi.org/10.1021/acsami.7b13909
87. Field J, Haycock JW, Boissonade FM, et al., 2021, A Tuneable, Photocurable, Poly(Caprolactone)-Based Resin for Tissue Engineering-Synthesis, Characterisation and Use in Stereolithography. Molecules, 26:1199. https://doi.org/10.3390/molecules26051199