Light-based and cost-effective bioprinting of musculoskeletal GelMA constructs enriched with mesoporous bioactive glass nanoparticles
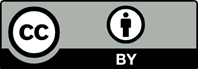
Bioprinting represents a promising technique for fabricating three-dimensional (3D) constructs with high-resolution and controlled architecture. However, many bioprinting technologies rely on expensive extrusion systems, which may compromise cell viability due to harsh processing conditions. This study presents the fabrication and characterization of musculoskeletal tissue in gelatin methacryloyl [GelMA]-based nanocomposite 3D constructs (comprising GelMA and mesoporous bioactive glass nanoparticles [MBGNs]) using a cost-effective, light-based bioprinting (LBB) system. We demonstrated that our strategy can produce high-resolution constructs (approximately 250 μm) while maintaining high cell viabilities (above 85%) for extended periods (weeks of culture). Furthermore, the nanocomposite constructs could facilitate the maturation of musculoskeletal tissue derived from C2C12 cells, as indicated by assessments of cell viability, elongation, and alignment over time. Our results suggested that the bioprinting approach outlined in this study allows for precise control of architecture, while creating a conducive environment for cell growth and tissue formation. These findings also highlighted the potential of the proposed LBB system to advance musculoskeletal tissue engineering for regenerative medicine applications.
- Downing K, Prisby R, Varanasi V, Zhou J, Pan Z, Brotto M. Old and new biomarkers for volumetric muscle loss. Curr Opin Pharmacol. 2021;59:61-69. doi: 10.1016/j.coph.2021.05.001
- McFaline-Figueroa J, Schifino AG, Nichenko AS, et al. Pharmaceutical agents for contractile-metabolic dysfunction after volumetric muscle loss. Tissue Eng Part A. 2022; 28(17-18):795-806. doi: 10.1089/ten.TEA.2022.0036
- Ahuja N, Awad K, Peper S, Brotto M, Varanasi V. Mini review: biomaterials in repair and regeneration of nerve in a volumetric muscle loss. Neurosci Lett. 2021;762: 136145. doi: 10.1016/j.neulet.2021.136145
- Tavares-Negrete JA, Pedroza-González SC, Frías-Sánchez AI, et al. Supplementation of GelMA with minimally processed tissue promotes the formation of densely packed skeletal-muscle-like tissues. ACS Biomater Sci Eng. 2023;9(6):3462-3475. doi: 10.1021/acsbiomaterials.2c01521
- Carnes ME, Pins GD. Skeletal muscle tissue engineering: biomaterials-based strategies for the treatment of volumetric muscle loss. Bioengineering (Basel). 2020;7(3):85. doi: 10.3390/bioengineering7030085
- Frías-Sánchez AI, Quevedo-Moreno DA, Samandari M, et al. Biofabrication of muscle fibers enhanced with plant viral nanoparticles using surface chaotic flows. Biofabrication. 2021;13(3). doi: 10.1088/1758-5090/abd9d7
- Bolívar-Monsalve EJ, Ceballos-González CF, Borrayo- Montaño KI, et al. Continuous chaotic bioprinting of skeletal muscle-like constructs. Bioprinting. 2021; 21:e00125. doi: 10.1016/j.bprint.2020.e00125
- Bolívar-Monsalve EJ, Ceballos-González CF, Chávez- Madero C, et al. One-step bioprinting of multi-channel hydrogel filaments using chaotic advection: fabrication of pre-vascularized muscle-like tissues. Adv Healthc Mater. 2022;11(24):e2200448. doi: 10.1002/adhm.202200448
- Zhuang P, An J, Chua CK, Tan LP. Bioprinting of 3D in vitro skeletal muscle models: a review. Maters Design. 2020;193:108794. doi: 10.1016/j.matdes.2020.108794
- Daly AC, Prendergast ME, Hughes AJ, Burdick JA. Bioprinting for the biologist. Cell. 2021;184(1):18-32. doi: 10.1016/j.cell.2020.12.002
- Samandari M, Quint J, Rodríguez-de la Rosa A, Sinha I, Pourquié O, Tamayol A. Bioinks and bioprinting strategies for skeletal muscle tissue engineering. Adv Mater. 2022;34(12):2105883. doi: 10.1002/adma.202105883
- Nieto D, Marchal Corrales JA, Jorge de Mora A, Moroni L. Fundamentals of light-cell–polymer interactions in photo-cross-linking based bioprinting. APL Bioeng. 2020;4(4):041502. doi: 10.1063/5.0022693
- Ege D, Hasirci V. Is 3D printing promising for osteochondral tissue regeneration? ACS Appl Bio Mater. 2023;6(4): 1431-1444. doi: 10.1021/acsabm.3c00093
- Arcaute K, Mann B, Wicker R. Stereolithography of spatially controlled multi-material bioactive poly(ethylene glycol) scaffolds. Acta Biomater. 2010;6(3):1047-1054. doi: 10.1016/j.actbio.2009.08.017
- Yi S, Liu Q, Luo Z, et al. Micropore-forming gelatin methacryloyl (gelma) bioink toolbox 2.0: designable tunability and adaptability for 3D bioprinting applications. Small. 2022;18(25):e2106357. doi: 10.1002/smll.202106357
- Levato R, Dudaryeva O, Garciamendez-Mijares CE, et al. Light-based vat-polymerization bioprinting. Nature Reviews Methods Primers. 2023;3(1):47. doi: 10.1038/s43586-023-00231-0
- Ying G, Jiang N, Yu C, Zhang YS. Three-dimensional bioprinting of gelatin methacryloyl (GelMA). Bio-des Manuf. 2018;1(4):215-224. doi: 10.1007/s42242-018-0028-8
- Mamidi N, Velasco Delgadillo RM, Barrera EV. Covalently functionalized carbon nano-onions integrated gelatin methacryloyl nanocomposite hydrogel containing γ-cyclodextrin as drug carrier for high-performance ph-triggered drug release. Pharmaceuticals (Basel).2021;14(4):291. doi: 10.3390/ph14040291
- Mamidi N, Villela Castrejón J, González-Ortiz A. Rational design and engineering of carbon nano-onions reinforced natural protein nanocomposite hydrogels for biomedical applications. J Mech Behav Biomed Mater. 2020;104: 103696. doi: 10.1016/j.jmbbm.2020.103696
- Daly AC, Critchley SE, Rencsok EM, Kelly DJ. A comparison of different bioinks for 3D bioprinting of fibrocartilage and hyaline cartilage. Biofabrication. 2016;8(4):045002. doi: 10.1088/1758-5090/8/4/045002
- Pedroza-González SC, Rodriguez-Salvador M, Pérez- Benítez BE, Alvarez MM, Trujillo-de Santiago G. Bioinks for 3D bioprinting: a scientometric analysis of two decades of progress. Int J Bioprint. 2021;7(2):333. doi: 10.18063/ijb.v7i2.337
- Yue K, Trujillo-de Santiago G, Alvarez MM, Tamayol A, Annabi N, Khademhosseini A. Synthesis, properties, and biomedical applications of gelatin methacryloyl (GelMA) hydrogels. Biomaterials. 2015;73:254-271. doi: 10.1016/j.biomaterials.2015.08.045
- Jones JR. Review of bioactive glass: from Hench to hybrids. Acta Biomater. 2013;9(1):4457-4486. doi: 10.1016/j.actbio.2012.08.023
- El-Fiqi A, Kim TH, Kim M, et al. Capacity of mesoporous bioactive glass nanoparticles to deliver therapeutic molecules. Nanoscale. 2012;4(23):7475-7488. doi: 10.1039/C2NR31775C
- El-Rashidy A, Roether J, Harhaus L, Kneser U, Boccaccini AR. Regenerating bone with bioactive glass scaffolds: a review of in vivo studies in bone defect models. Acta Biomater. 2017;62:1-28. doi: 10.1016/j.actbio.2017.08.030
- Hasan A, Morshed M, Memic A, Hassan S, Webster TJ, Marei HES. Nanoparticles in tissue engineering: applications, challenges and prospects. Int J Nanomedicine. 2018;13: 5637-5655. doi: 10.2147/IJN.S153758
- Chen QZ, Thompson ID, Boccaccini AR. 45S5 Bioglass®- derived glass–ceramic scaffolds for bone tissue engineering. Biomaterials. 2006;27(11):2414-2425. doi: 10.1016/j.biomaterials.2005.11.025
- Jia W, Hu H, Li A, et al. Glass-activated regeneration of volumetric muscle loss. Acta Biomater. 2020;103:306-317. doi: 10.1016/j.actbio.2019.12.007
- Ege D, Nawaz Q, Beltrán AM, Boccaccini AR. Effect of boron-doped mesoporous bioactive glass nanoparticles on c2c12 cell viability and differentiation: potential for muscle tissue application. ACS Biomater Sci Eng. 2022;8(12): 5273-5283. doi: 10.1021/acsbiomaterials.2c00876
- Winston DD, Li T, Lei B. Bioactive nanoglass regulating the myogenic differentiation and skeletal muscle regeneration. Regenerative Biomater. 2023;10:rbad059. doi: 10.1093/rb/rbad059
- Rahaman MN, Day DE, Bal BS, et al. Bioactive glass in tissue engineering. Acta Biomater. 2011;7(6):2355-2373. doi: 10.1016/j.actbio.2011.03.016
- Ceballos-González CF, Bolívar Monsalve EJ, Quevedo‐ Moreno DA, et al. Plug‐and‐play multimaterial chaotic printing/bioprinting to produce radial and axial micropatterns in hydrogel filaments. Adv Mater Technol. 2023;8(17):202202208. doi: 10.1002/admt.202202208
- Yang X, Dargaville BL, Hutmacher DW. Elucidating the molecular mechanisms for the interaction of water with polyethylene glycol-based hydrogels: influence of ionic strength and gel network structure. Polymers (Basel). 2021;13(6):845. doi: 10.3390/polym13060845
- Vigata M, Meinert C, Bock N, Dargaville BL, Hutmacher DW. Deciphering the molecular mechanism of water interaction with gelatin methacryloyl hydrogels: role of ionic strength, ph, drug loading and hydrogel network characteristics. Biomedicines. 2021;9(5):574. doi: 10.3390/biomedicines9050574
- Pérez Cortez JE, Sánchez-Rodríguez VH, Vázquez E, Trujillo-de Santiago G, Alvarez MM, Martínez-López JI. Retrofitting of an affordable 3D printer: towards a material efficient and low-cost bioprinting system. Procedia CIRP. 2022;110:150-155. doi: 10.1016/j.procir.2022.06.028
- Pérez-Cortez JE, Sánchez-Rodríguez VH, Gallegos- Martínez S, et al. Low-cost light-based GelMA 3D bioprinting via retrofitting: manufacturability test and cell culture assessment. Micromachines (Basel). 2022; 14(1):55. doi: 10.3390/mi14010055
- Font Tellado S, Balmayor ER, Van Griensven M. Strategies to engineer tendon/ligament-to-bone interface: biomaterials, cells and growth factors. Adv Drug Deliv Rev. 2015; 94:126-140. doi: 10.1016/j.addr.2015.03.004
- Gao L, Zhou Y, Peng J, et al. A novel dual-adhesive and bioactive hydrogel activated by bioglass for wound healing. NPG Asia Mater. 2019;11(1):66. doi: 10.1038/s41427-019-0168-0
- Zöllner AM, Abilez OJ, Böl M, Kuhl E. Stretching skeletal muscle: chronic muscle lengthening through sarcomerogenesis. PLoS One. 2012;7(10): e45661. doi: 10.1371/journal.pone.0045661
- Vasita R. A review on extracellular matrix mimicking strategies for an artificial stem cell niche. Polym Rev. 2015;55(4):561-595. doi: 10.1080/15583724.2015.1040552
- Yin H, Price F, Rudnicki MA. Satellite cells and the muscle stem cell niche. Physiol Rev. 2013;93(1):23-67. doi: 10.1152/physrev.00043.2011
- Relaix F, Zammit PS. Satellite cells are essential for skeletal muscle regeneration: the cell on the edge returns centre stage. Development. 2012;139(16):2845-2856. doi: 10.1242/dev.069088
- Heinemeier KM, Schjerling P, Heinemeier J, Magnusson SP, Kjaer M. Lack of tissue renewal in human adult Achilles tendon is revealed by nuclear bomb 14C. FASEB J. 2013;27(5):2074-2079. doi: 10.1096/fj.12-225599
- Ogneva IV, Lebedev DV, Shenkman BS. Transversal stiffness and Young’s modulus of single fibers from rat soleus muscle probed by atomic force microscopy. Biophys J. 2010;98(3):418-424. doi: 10.1016/j.bpj.2009.10.028
- Collinsworth AM, Zhang S, Kraus WE, Truskey GA. Apparent elastic modulus and hysteresis of skeletal muscle cells throughout differentiation. Am J Physiol Cell Physiol. 2002;283(4):C1219-C1227. doi: 10.1152/ajpcell.00502.2001
- Elvitigala KCML, Mubarok W, Sakai S. Tuning the crosslinking and degradation of hyaluronic acid/gelatin hydrogels using hydrogen peroxide for muscle cell sheet fabrication. Soft Matter. 2023;19(31):5880-5887. doi: 10.1039/D3SM00560G
- Gao H, Xiao J, Wei Y, Wang H, Wan H, Liu S. Regulation of myogenic differentiation by topologically microgrooved surfaces for skeletal muscle tissue engineering. ACS Omega. 2021;6(32):20931-20940. doi: 10.1021/acsomega.1c02347
- Wu C, Chin CSM, Huang Q, et al. Rapid nanomolding of nanotopography on flexible substrates to control muscle cell growth with enhanced maturation. Microsyst Nanoeng. 2021;7(1):1-15. doi: 10.1038/s41378-021-00316-4
- Yu K, Zhang X, Sun Y, et al. Printability during projection-based 3D bioprinting. Bioact Mater. 2022;11:254-267. doi: 10.1016/j.bioactmat.2021.09.021