Effect of sterilization processes on alginate/gelatin inks for three-dimensional printing
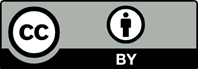
Sterilization is a crucial step in the process of developing bioinks for tissue engineering applications. In this work, alginate/gelatin inks were subjected to three sterilization methods: ultraviolet (UV) radiation, filtration (FILT), and autoclaving (AUTO). In addition, to simulate the sterilization effect in a real environment, inks were formulated in two different media, specifically, Dulbecco’s Modified Eagle’s Medium (DMEM) and phosphate-buffered saline (PBS). First, rheological tests were performed to evaluate the flow properties of the inks, and we observed that UV samples showed shear thinning behavior, which was favorable for threedimensional (3D) printing. Furthermore, the 3D-printed constructs developed with UV inks showed better shape and size fidelity than those obtained with FILT and AUTO. In order to relate this behavior to the material structure, Fourier transform infrared (FTIR) analysis was carried out and the predominant conformation in protein was determined by deconvolution of the amide I band, which confirmed that the prevalence of a-helix structure was greater for UV samples. This work highlights the relevance of sterilization processes, which are essential for biomedical applications, in the research field of bioinks.
1. Lopes Nalesso PR, Wang W, Hou Y, et al., 2021, In vivo investigation of 3D printed polycaprolactone/graphene electro-active bone scaffolds. Bioprinting, 24: e00164.
2. Liu X, Xu M, Li P, et al., 2021, Implantation and repair of 3D printed myocardial patch in rabbit model of myocardial infarction. Bioprinting, 24: e00165.
3. Maxson EL, Young MD, Noble C, et al., 2019, In vivo remodeling of a 3D-Bioprinted tissue engineered heart valve scaffold. Bioprinting, 16: e00059.
4. Tian S, Zhao H, Lewinski N, 2021, Key parameters and applications of extrusion-based bioprinting. Bioprinting, 23: e00156.
5. Dutta SD, Hexiu J, Patel DK, et al., 2021, 3D-printed bioactive and biodegradable hydrogel scaffolds of alginate/gelatin/cellulose nanocrystals for tissue engineering. Int J Biol Macromol, 167: 644–658.
6. He Y, Yang F, Zhao H, et al., 2016, Research on the printability of hydrogels in 3D bioprinting. Sci Rep, 6: 29977.
7. Horakova J, Klicova M, Erben J, et al., 2020, Impact of various sterilization and disinfection techniques on electrospun poly-ε-caprolactone. ACS Omega, 5: 8885–8892.
8. Dai Z, Ronholm J, Tian Y, et al., 2016, Sterilization techniques for biodegradable scaffolds in tissue engineering applications. J Tissue Eng, 7: 2041731416648810.
9. Stoppel WL, White JC, Horava SD, et al., 2014, Terminal sterilization of alginate hydrogels: Efficacy and impact on mechanical properties. J Biomed Mater Res B Appl Biomater, 102: 877–884.
10. Fidalgo C, Iop L, Sciro M, et al., 2018, A sterilization method for decellularized xenogeneic cardiovascular scaffolds. Acta Biomater, 67: 282–294.
11. Hofmann S, Stok KS, Kohler T, et al., 2014, Effect of sterilization on structural and material properties of 3-D silk fibroin scaffolds. Acta Biomater, 10: 308–317.
12. Munarin F, Bozzini S, Visai L, et al., 2013, Sterilization treatments on polysaccharides: Effects and side effects on pectin. Food Hydrocoll, 31: 74–84.
13. Galante R, Pinto TJA, Colaço R, et al., 2018, Sterilization of hydrogels for biomedical applications: A review. J Biomed Mater Res B Appl Biomater, 106: 2472–2492.
14. Ribeiro N, Soares GC, Santos-Rosales V, et al., 2020, A new era for sterilization based on supercritical CO2 technology. J Biomed Mater Res B Appl Biomater, 108: 399–428.
15. Di Foggia M, Corda U, Plescia E, et al., 2010, Effects of sterilisation by high-energy radiation on biomedical poly-(ε-caprolactone)/hydroxyapatite composites. J Mater Sci: Mater Med, 21: 1789–97.
16. Bernhardt A, Wehrl M, Paul B, et al., 2015, Improved sterilization of sensitive biomaterials with supercritical carbon dioxide at low temperature. PLoS ONE, 10: e0129205.
17. Hoang VT, Stępniewski G, Czarnecka KH, et al., 2019, Optical properties of buffers and cell culture media for optofluidic and sensing applications. Appl Sci, 9: 1145.
18. Puertas-Bartolomé M, Włodarczyk-Biegun MK, del Campo A, et al., 2020, 3D printing of a reactive hydrogel bio-ink using a static mixing tool. Polymers, 12: 1986.
19. Lorson T, Ruopp M, Nadernezhad A, et al., 2020, Sterilization methods and their influence on physicochemical properties and bioprinting of alginate as a bioink component. ACS Omega, 5: 6481–6486.
20. O’Connell CD, Onofrillo C, Duchi S, et al., 2019, Evaluation of sterilisation methods for bio-ink components: Gelatin, gelatin methacryloyl, hyaluronic acid and hyaluronic acid methacryloyl. Biofabrication, 11: 035003.
21. Chansoria P, Narayanan LK, Wood M, et al., 2020, Effects of autoclaving, etoh, and uv sterilization on the chemical, mechanical, printability, and biocompatibility characteristics of alginate. ACS Biomater Sci Eng, 6: 5191–5201.
22. Rizwan M, Chan SW, Comeau PA, et al., 2020, Effect of sterilization treatment on mechanical properties, biodegradation, bioactivity and printability of GelMA hydrogels. Biomed Mater, 15: 065017.
23. Lafuente-Merchan M, Ruiz-Alonso S, Espona-Noguera A, et al., 2021, Development, characterization and sterilisation of Nanocellulose-alginate-(hyaluronic acid)- bioinks and 3D bioprinted scaffolds for tissue engineering. Mater Sci Eng C, 126: 112160.
24. Pan T, Song W, Cao X, 2016, 3D bioplotting of gelatin/alginate scaffolds for tissue engineering: influence of crosslinking degree and pore architecture on physicochemical properties. J Mater Sci Technol, 32: 889–900.
25. Merk M, Chirikian O, Adlhart C, 2021, 3D PCL/gelatin/genipin nanofiber sponge as scaffold for regenerative medicine. Materials, 14: 2006.
26. Li H, Huang C, Jin X, et al., 2018, An electrospun poly(ε- caprolactone) nanocomposite fibrous mat with a high content of hydroxyapatite to promote cell infiltration RSC Adv, 8: 25228–25235.
27. Choi DJ, Park SJ, Gu BK, et al., 2018, Effect of the pore size in a 3D bioprinted gelatin scaffold on fibroblast proliferation. J Ind Eng Chem, 67: 388–395.
28. Xu J, Fang H, Su Y, et al., 2022, A 3D bioprinted decellularized extracellular matrix/gelatin/quaternized chitosan scaffold assembling with poly(ionic liquid)s for skin tissue engineering. Int J Biol Macromol, 220: 1253–1266.
29. Ren P, Wei D, Liang M, et al., 2022, Alginate/gelatin based hybrid hydrogels with function of injecting and encapsulating cells in situ. Int J Biol Macromol, 212: 67–84.
30. Wu Y, Lin Z Y (William), Wenger AC, et al., 2018, 3D bioprinting of liver-mimetic construct with alginate/ cellulose nanocrystal hybrid bioink. Bioprinting, 9: 1–6.
31. Russell CS, Mostafavi A, Quint JP, et al., 2020, In situ printing of adhesive hydrogel scaffolds for the treatment of skeletal muscle injuries. ACS Appl Bio Mater, 3: 1568–1579.
32. Hamid OA, Eltaher HM, Sottile V, et al., 2021, 3D bioprinting of a stem cell-laden, multi-material tubular composite: An approach for spinal cord repair. Mater Sci Eng C, 120: 111707.
33. Hooper R, Arish AA, Alejandre RT, et al., 2022, Chaotic printing of hydrogel carriers for human mesenchymal stem cell expansion. Procedia CIRP, 110: 236–241.
34. Fayyabakhsh F, Khayat MJ, Leu MC, 2022, 3D-printed gelatin-alginate hydrogel dressings for burn wound healing: A comprehensive study. Int J Bioprint, 8. 274–291.
35. Reis DP, Domingues B, Fidalgo C, et al., 2022, Bioinks enriched with ecm components obtained by supercritical extraction. Biomolecules, 12: 394.
36. Pössl A, Hartzke D, Schmidts TM, et al., 2021, A targeted rheological bioink development guideline and its systematic correlation with printing behavior. Biofabrication, 13: 035021.
37. Calafel I, Aguirresarobe RH, Peñas MI, et al., 2020, Searching for rheological conditions for FFF 3D Printing with PVC based flexible compounds. Materials, 13: 178.
38. Han CD, 1976, Rheology in Polymer Processing, Academic Press, New York.
39. Sanchez LC, Beatrice C AG, Lotti C, et al., 2019, Rheological approach for an additive manufacturing printer based on material extrusion. Int J Adv Manuf Technol, 105: 2403–2414.
40. Bom S, Ribeiro R, Ribeiro HM, et al., 2022, On the progress of hydrogel-based 3D printing: Correlating rheological properties with printing behaviour. Int J Pharm, 615: 121506.
41. Amorim PA, d’Ávila MA, Anand R, et al., 2021, Insights on shear rheology of inks for extrusion-based 3D bioprinting. Bioprinting, 22: e00129.
42. Naranda J, Bračič M, Vogrin M, et al., 2021, Recent advancements in 3D printing of polysaccharide hydrogels in cartilage tissue engineering. Materials, 14: 3977.
43. Dodero A, Vicini S, Alloisio M, et al., 2020, Rheological properties of sodium alginate solutions in the presence of added salt: an application of Kulicke equation. Rheol Acta, 59: 365–374.
44. Lee SC, Gillispie G, Prim P, et al., 2020, Physical and chemical factors influencing the printability of hydrogel based extrusion bioinks. Chem Rev, 120: 10834–10886.
45. Habib MA, Khoda B, 2022, Rheological analysis of bio-ink for 3D bio-printing processes. J Manuf Process, 76: 708–718.
46. Schwab A, Levato R, D’Este M, et al., 2020, Printability and shape fidelity of bioinks in 3D bioprinting. Chem Rev, 120: 11028–11055.
47. Chen H, Fei F, Li X, et al., 2021, A facile, versatile hydrogel bioink for 3D bioprinting benefits long-term subaqueous fidelity, cell viability and proliferation. Regen Biomater, 8: rbab026.
48. Gómez-Blanco JC, Mancha-Sánchez E, Marcos AC, et al., 2020, Bioink Temperature influence on shear stress, pressure and velocity using computational simulation. Processes, 8: 865.
49. Ke C-J, Chiu K-H, Chen C-Y, et al., 2021, Alginate-gelatin based core-shell capsule enhances the osteogenic potential of human osteoblast-like MG-63 cells. Materials & Design, 210: 110109.
50. Steven E, Saleh WR, Lebedev V, et al., 2013, Carbon nanotubes on a spider silk scaffold. Nat Commun, 4: 2435.
51. Yang H, Yang S, Kong J, et al., 2015, Obtaining information about protein secondary structures in aqueous solution using Fourier transform IR spectroscopy. Nat Protoc, 10: 382–396.
52. Fellows AP, Casford MTL, Davies PB, 2020, Spectral analysis and deconvolution of the amide I band of proteins presenting with high-frequency noise and baseline shifts. Appl Spectrosc, 74: 597–615.
53. Ladner-Keay CL, Griffith BJ, Wishart DS, 2014, Shaking alone\ induces de novo conversion of recombinant prion proteins to β-sheet rich oligomers and fibrils. PLOS ONE, 9: e98753.
54. Guerrero P, Garrido T, Garcia-Orue I, et al., 2021, Characterization of bio-inspired electro-conductive soy protein films. Polymers, 13: 416.