Development of a five-axis printer for the fabrication of hybrid 3D scaffolds: From soft to hard phases and planar to curved surfaces
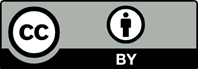
Three-dimensional (3D) printing of hybrid scaffolds with material gradients, combining soft and hard phases, is an appealing frontier in additive manufacturing. However, most 3D printers are limited to either three-axis or mono-material capabilities, rendering them unsuitable for fabricating hybrid scaffolds. Additionally, printing on curved surfaces requires advanced printing capabilities. Our work aims to advance additive manufacturing by developing a hybrid piezoelectric inkjet-extrusion printer equipped with five-axis functionalities. The printer could be used to fabricate customized hybrid scaffolds, surpassing conventional mono-material or linear three-axis printing strategies. The soft phase comprises a low-viscosity photocurable resin and a high-viscosity peptide hydrogel, while the hard phase comprises 3D-printed polylactic acid and hydroxyapatite parts. To validate the system, we fabricated three hybrid scaffolding use cases, characterized by multi-material porous structures fabricated on planar, single-curved, and free-form surfaces. The scaffolds were subsequently analyzed using digital microscopy to assess their accuracy, particularly the feature sizes of pores and struts (i.e., 0.8–3.6 mm). In the first part of the study, we demonstrated the versatility of inkjet and extrusion printing by hybrid printing an interconnected network in the soft phase on top of a planar ceramic hard phase. A pore width and height deviation of 6% was achieved compared to the intended design. In the second part of the study, we evaluated the 3D inkjet printing of a multi-material porous scaffold on a single-curved surface for osteochondral defects. The circumferential pore width and radial pore height deviated by 0.8% and 2%, respectively. Finally, we inkjet-printed a mesh structure on a free-form surface, which acted as a membrane for palatal implants. In this case, the pore width deviations were -16% in the printing direction and 2% perpendicular to the printing direction.
- Gleadall A, Visscher D, Yang J, Thomas D, Segal J. Review of additive manufactured tissue engineering scaffolds: relationship between geometry and performance. Burns Trauma. 2018;6:19. doi: 10.1186/s41038-018-0121-4
- Seidi A, Ramalingam M, Elloumi-Hannachi I, Ostrovidov S, Khademhosseini A. Gradient biomaterials for soft-to-hard interface tissue engineering. Acta Biomater. 2011;7(4): 1441-1451. doi: 10.1016/j.actbio.2011.01.011
- Wang C, Huang W, Zhou Y, et al. 3D printing of bone tissue engineering scaffolds. Bioact Mater. 2020;5(1):82-91. doi: 10.1016/j.bioactmat.2020.01.004
- Truby RL, Lewis JA. Printing soft matter in three dimensions. Nature. 2016;540(7633):371-378. doi: 10.1038/nature21003
- Haglin JM, Eltorai AEM, Gil JA, Marcaccio SE, Botero- Hincapie J, Daniels AH. Patient-specific orthopaedic implants. Orthop Surg. 2016;8(4):417-424. doi: 10.1111/os.12282
- Bittner SM, Guo JL, Melchiorri A, Mikos AG. Three-dimensional printing of multilayered tissue engineering scaffolds. Mater Today. 2018;21(8):861-874. doi: 10.1016/J.MATTOD.2018.02.006
- Taboas JM, Maddox RD, Krebsbach PH, Hollister SJ. Indirect solid free form fabrication of local and global porous, biomimetic and composite 3D polymer-ceramic scaffolds. Biomaterials. 2003;24(1):181-194. doi: 10.1016/S0142-9612(02)00276-4
- Lantada AD, Iniesta HA, Garcia-Ruiz JP. Composite scaffolds for osteochondral repair obtained by combination of additive manufacturing, leaching processes and hMSC-CM functionalization. Mater Sci Eng C. 2016;59:218-227. doi: 10.1016/j.msec.2015.10.015
- Sänger JC, Schwentenwein M, Bermejo R, Günster J. Hybridizing lithography-based ceramic additive manufacturing with two-photon-polymerization. Appl Sci (Basel). 2023;13(6). doi: 10.3390/app13063974
- Gonzalez-Pujana A, Carranza T, Santos-Vizcaino E, et al. Hybrid 3D printed and electrospun multi-scale hierarchical polycaprolactone scaffolds to induce bone differentiation. Pharmaceutics. 2022;14(12). doi: 10.3390/pharmaceutics14122843
- Choi WS, Kim JH, Ahn CB, et al. Development of a multi-layer skin substitute using human hair keratinic extract-based hybrid 3D printing. Polymers (Basel). 2021;13(16):2584. doi: 10.3390/polym13162584
- Altunbek M, Afghah SF, Fallah A, Acar AA, Koc B. Design and 3D printing of personalized hybrid and gradient structures for critical size bone defects. ACS Appl Bio Mater. 2023;6(5):1873-1885. doi: 10.1021/acsabm.3c00107
- Milojević M, Harih G, Vihar B, et al. Hybrid 3D printing of advanced hydrogel-based wound dressings with tailorable properties. Pharmaceutics. 2021;13(4):564. doi: 10.3390/pharmaceutics13040564
- Stögerer J, Baumgartner S, Hochwallner A, Stampfl J. Bio-inspired toughening of composites in 3D-printing. Materials (Basel). 2020;13(21). doi: 10.3390/ma13214714
- Lee HR, Park JA, Kim S, Jo Y, Kang D, Jung S. 3D microextrusion-inkjet hybrid printing of structured human skin equivalents. Bioprinting. 2021;22:e00143. doi: 10.1016/j.bprint.2021.e00143
- Jaksa L, Pahr D, Kronreif G, Lorenz A. Development of a multi-material 3D printer for functional anatomic models. Int J Bioprint. 2021;7(4):420. doi: 10.18063/ijb.v7i4.420
- Tashman JW, Shiwarski DJ, Feinberg AW. Development of a high-performance open-source 3D bioprinter. Sci Rep. 2022;12(1):22652. doi: 10.1038/s41598-022-26809-4
- Hinton TJ, Jallerat Q, Palchesko RN, et al. Three-dimensional printing of complex biological structures by freeform reversible embedding of suspended hydrogels. Sci Adv. 2015;1(9):e1500758. doi: 10.1126/sciadv.1500758
- Pusch K, Hinton TJ, Feinberg AW. Large volume syringe pump extruder for desktop 3D printers. HardwareX. 2018;3:49-61. doi: 10.1016/j.ohx.2018.02.001
- Mirdamadi E, Tashman JW, Shiwarski DJ, Palchesko RN, Feinberg AW. FRESH 3D bioprinting a full-size model of the human heart. ACS Biomater Sci Eng. 2020;6(11): 6453-6459. doi: 10.1021/acsbiomaterials.0c01133
- Khani N, Nadernezhad A, Bartolo P, Koc B. Hierarchical and spatial modeling and bio-additive manufacturing of multi-material constructs. CIRP Annals. 2017;66(1):229-232. doi: 10.1016/j.cirp.2017.04.132
- Hong F, Hodges S, Myant C, Boyle DE. Open5x: accessible 5-axis 3D printing and conformal slicing. Ext Abstr Hum Factors Computing Syst. 2022. doi: 10.1145/3491101.3519782
- Sheng YT, Liong S, Wang SY, Gan YS. 3D printing on freeform surface: real-time and accurate 3D dynamic dense surface reconstruction with HoloLens and displacement measurement sensors. Adv Mech Eng. 2023;15(1):168781322211484. doi: 10.1177/16878132221148404
- Arango I, Cifuentes C. Design to achieve accuracy in ink-jet cylindrical printing machines. Machines. 2019;7(1):6. doi: 10.3390/machines7010006
- Arango I, Bonil L, Posada D, Arcila J. Prediction of a flying droplet landing over a non-flat substrates for ink-jet applications. Int J Interact Des Manuf. 2019;13:967-980 doi: 10.1007/s12008-019-00547-w
- Fechtig D. Robot-based direct digital printing on freeform surfaces. In: Zapka W, ed. Inkjet Printing in Industry. Weinheim: Wiley-VCH; 2022:1269-1297. doi: 10.1002/9783527828074.CH55
- Thalheim R, Willert A, Mitra D, Zichner R. Novel and efficient methodology for drop placement accuracy testing of robot-guided inkjet printing onto 3D objects. Machines. 2023;11(5):568. doi: 10.3390/machines11050568
- Shen H, Liu B, Liu S, Fu J. Five-axis freeform surface color printing technology based on offset curve path planning method. Appl Sci (Basel). 2020;10(5):1716. doi: 10.3390/app10051716
- Gazeau JP, Said Z, Ramírez-Torres J. A novel 5-axis robot for printing high resolution pictures from media on 3D wide surfaces. Proceedings of the IEEE International Conference on Industrial Technology. 2009:1-6. doi: 10.1109/ICIT.2009.4939735
- Urasinska-Wojcik B, Chilton N, Todd P, et al. Integrated manufacture of polymer and conductive tracks for real-world applications. Addit Manuf. 2019;29:100777. doi: 10.1016/j.addma.2019.06.028
- Moroni L, Boland T, Burdick JA, et al. Biofabrication: a guide to technology and terminology. Trends Biotechnol. 2018;36(4):384-402. doi: 10.1016/j.tibtech.2017.10.015
- Kainz M, Perak S, Stubauer G, et al. Additive and lithographic manufacturing of biomedical scaffold structures using a versatile thiol-ene photocurable resin. Polymers (Basel). 2024;16(5):655. doi: 10.3390/polym16050655
- Arosio P, Owczarz M, Wu H, Butté A, Morbidelli M. End-to-end self-assembly of RADA 16-I nanofibrils in aqueous solutions. Biophys J. 2012;102(7): 1617-1626. doi: 10.1016/j.bpj.2012.03.012
- Sankar S, O’Neill K, Bagot D’Arc M, et al. Clinical use of the self-assembling peptide RADA16: a review of current and future trends in biomedicine. Front Bioeng Biotechnol. 2021;9. doi: 10.3389/fbioe.2021.679525
- Wong KC. 3D-printed patient-specific applications in orthopedics. Orthop Res Rev. 2016;8:57-66. doi: 10.2147/ORR.S99614
- Mobbs RJ, Coughlan M, Thompson R, Sutterlin CE, Phan K. The utility of 3D printing for surgical planning and patient-specific implant design for complex spinal pathologies: case report. J Neurosurg. 2017;26(4):513-518. doi: 10.13140/RG.2.2.18087.75686
- Lawrence S. Developable surfaces: their history and application. Nexus Netw J. 2011;13(3):701-714. doi: 10.1007/s00004-011-0087-z
- Baselga S, Olsen M. Approximations, errors, and misconceptions in the use of map projections. Math Probl Eng. 2021;2021:1-12. doi: 10.1155/2021/1094602
- Roach BL, Hung CT, Cook JL, Ateshian GA, Tan AR. Fabrication of tissue engineered osteochondral grafts for restoring the articular surface of diarthrodial joints. Methods. 2015;84:103-108. doi: 10.1016/j.ymeth.2015.03.008
- Woodfield TBF, Guggenheim M, von Rechenberg B, Riesle J, van Blitterswijk CA, Wedler V. Rapid prototyping of anatomically shaped, tissue-engineered implants for restoring congruent articulating surfaces in small joints. Cell Prolif. 2009;42(4):485-497. doi: 10.1111/j.1365-2184.2009.00608.x
- Guilak F, Estes BT, Moutos FT. Functional tissue engineering of articular cartilage for biological joint resurfacing-The 2021 Elizabeth Winston Lanier Kappa Delta Award. J Orthop Res. 2022;40(8):1721-1734. doi: 10.1002/jor.25223
- Kilian D, Ahlfeld T, Akkineni AR, Bernhardt A, Gelinsky M, Lode A. 3D bioprinting of osteochondral tissue substitutes – in vitro-chondrogenesis in multi-layered mineralized constructs. Sci Rep. 2020;10(1):8277. doi: 10.1038/s41598-020-65050-9
- Fudalej P, Katsaros C, Dudkiewicz Z, et al. Dental arch relationships following palatoplasty for cleft lip and palate repair. J Dent Res. 2012;91(1):47-51. doi: 10.1177/0022034511425674
- Figueroa AA, Murphy J, Tragos C. Intra-lesional injection of triamcinolone to palatoplasty scar to aid reversal of transverse maxillary relapse after orthognathic surgery. J Craniofac Surg. 2022;33(4):e416-e418. doi: 10.1097/SCS.0000000000008347
- Ren Y, Fan L, Alkildani S, et al. Barrier membranes for guided bone regeneration (GBR): a focus on recent advances in collagen membranes. Int J Mol Sci. 2022;23(23):14987. doi: 10.3390/ijms232314987
- Tayebi L, Rasoulianboroujeni M, Moharamzadeh K, Almela TKD, Cui Z, Ye H. 3D-printed membrane for guided tissue regeneration. Mater Sci Eng C. 2018;84: 148-158. doi: 10.1016/j.msec.2017.11.027
- Vahdatinia F, Hooshyarfard A, Jamshidi S, et al. 3D-printed soft membrane for periodontal guided tissue regeneration. Materials (Basel). 2023;16(4). doi: 10.3390/ma16041364
- Jang TS, Park SJ, Lee JE, et al. Topography-supported nanoarchitectonics of hybrid scaffold for systematically modulated bone regeneration and remodeling. Adv Funct Mater. 2022;32:2206863. doi: 10.1002/adfm.202206863
- Liang J, Zeng H, Qiao L, et al. 3D printed piezoelectric wound dressing with dual piezoelectric response models for scar-prevention wound healing. ACS Appl Mater Interfaces. 2022;14(27):30507-30522. doi: 10.1021/acsami.2c04168