Most utilized rodent models for Alzheimer’s and Parkinson’s disease: A critical review of the past 5 years
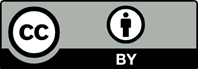
The past few years have witnessed extensive research on the two most common neurodegenerative diseases, Alzheimer’s disease (AD), and Parkinson’s disease (PD). With an urgent need for new treatments, drug targets, and a better understanding of the pathophysiological mechanisms underlying these conditions, researchers have turned to animal models, especially rodents, to address these issues. However, the abundance of reported models poses a challenge when choosing the most suitable model for a specific study. In this critical review, we systematically scrutinized studies using rodent models of AD or PD over the past 5 years. A comprehensive literature search was conducted on PubMed, followed by the meticulous screening of the identified studies. Among the retrieved publications, 1,222 studies reported the use of rodent models of PD, with 1-methyl-4-phenyl-1,2,3,6-tetrahydropyridine, α-synuclein, and 6-hydroxydopamine emerging as the most frequently used models. Similarly, 2,961 studies reported the use of rodent models of AD, with APP/PS1, 5×FAD, APP-based models, and 3×Tg being the most prevalent. In this review, we summarize and highlight the main characteristics of these models. By providing a comprehensive overview of their features and applications, this review guides future studies in the AD and PD field, eventually aiding in the selection of the most appropriate animal model tailored to the specific research question under scrutiny.
- United Nations. World Social Report 2023: Leaving No One Behind in an Ageing World. (Department of Economic and Social Affairs (DESA) PD, ed). United Nations; 2023. Available from: https://social.desa.un.org/sites/default/ files/publications/2023-02/worldsocialreport2023.pdf [Last accessedon 2024 Apr 14.
- Nichols E, Szoeke CEI, Vollset SE, et al. Global, regional, and national burden of Alzheimer’s disease and other dementias, 1990–2016: A systematic analysis for the Global Burden of Disease Study 2016. Lancet Neurol. 2019;18(1):88-106. doi: 10.1016/S1474-4422(18)30403-4
- Ou Z, Pan J, Tang S, et al. Global trends in the incidence, prevalence, and years lived with disability of Parkinson’s disease in 204 countries/territories from 1990 to 2019. Front Public Health. 2021;9:776847. doi: 10.3389/fpubh.2021.776847
- Denver P, McClean P. Distinguishing normal brain aging from the development of Alzheimer’s disease: Inflammation, insulin signaling and cognition. Neural Regen Res. 2018;13(10):1719. doi: 10.4103/1673-5374.238608
- Arosio P. Biophysical aspects of Alzheimer’s disease: Implications for pharmaceutical sciences : Theme: Drug discovery, development and delivery in Alzheimer’s disease guest editor: Davide Brambilla. Pharm Res. 2017;34(12):2628-2636. doi: 10.1007/s11095-017-2266-4
- DeTure MA, Dickson DW. The neuropathological diagnosis of Alzheimer’s disease. Mol Neurodegener. 2019;14(1):32. doi: 10.1186/s13024-019-0333-5
- Xu YJ, Au NPB, Ma CHE. Functional and phenotypic diversity of microglia: Implication for microglia-based therapies for Alzheimer’s disease. Front Aging Neurosci. 2022;14:896852. doi: 10.3389/fnagi.2022.896852
- Murayama S, Saito Y. Neuropathological diagnostic criteria for Alzheimer’s disease. Neuropathology. 2004;24(3):254-260. doi: 10.1111/j.1440-1789.2004.00571.x
- Poewe W, Seppi K, Tanner CM, et al. Parkinson disease. Nat Rev Dis Prim. 2017;3:17013. doi: 10.1038/nrdp.2017.13
- Hoehn MM, Yahr MD. Parkinsonism: Onset, progression and mortality. Neurology. 1967;17(5):427-442. doi: 10.1212/wnl.17.5.427
- Poewe W. Non-motor symptoms in Parkinson’s disease. Eur J Neurol. 2008;15 Suppl 1:14-20. doi: 10.1111/j.1468-1331.2008.02056.x
- Obeso JA, Rodríguez-Oroz MC, Rodríguez M, et al. Pathophysiology of the basal ganglia in Parkinson’s disease. Trends Neurosci. 2000;23(10 Suppl):S8-S19. doi: 10.1016/s1471-1931(00)00028-8
- Kinter LB, DeHaven R, Johnson DK, DeGeorge JJ. A brief history of use of animals in biomedical research and perspective on non-animal alternatives. ILAR J. 2021;62(1-2):7-16. doi: 10.1093/ilar/ilab020
- Bryda EC. The mighty mouse: The impact of rodents on advances in biomedical research. Mo Med. 2013;110(3):207-211.
- Dawson TM, Golde TE, Lagier-Tourenne C. Animal models of neurodegenerative diseases. Nat Neurosci. 2018;21(10):1370-1379. doi: 10.1038/s41593-018-0236-8
- Mustapha M, Mat Taib CN. MPTP-induced mouse model of Parkinson’s disease: A promising direction of therapeutic strategies. Bosn J Basic Med Sci. 2021;21(4):422-433. doi: 10.17305/bjbms.2020.5181
- Langston JW. The MPTP story. J Parkinsons Dis. 2017;7(S1):S11-S19. doi: 10.3233/JPD-179006
- Dauer W, Przedborski S. Parkinson’s disease. Neuron. 2003;39(6):889-909. doi: 10.1016/S0896-6273(03)00568-3
- Bhurtel S, Katila N, Srivastav S, Neupane S, Choi DY. Mechanistic comparison between MPTP and rotenone neurotoxicity in mice. Neurotoxicology. 2019;71:113-121. doi: 10.1016/j.neuro.2018.12.009
- Burré J. The synaptic function of α-synuclein. J Parkinsons Dis. 2015;5(4):699-713. doi: 10.3233/JPD-150642
- Karampetsou M, Ardah MT, Semitekolou M, et al. Phosphorylated exogenous alpha-synuclein fibrils exacerbate pathology and induce neuronal dysfunction in mice. Sci Rep. 2017;7(1):16533. doi: 10.1038/s41598-017-15813-8
- Gómez-Benito M, Granado N, García-Sanz P, Michel A, Dumoulin M, Moratalla R. Modeling Parkinson’s disease with the alpha-synuclein protein. Front Pharmacol. 2020;11:356. doi: 10.3389/fphar.2020.00356
- Harms AS, Delic V, Thome AD, et al. α-Synuclein fibrils recruit peripheral immune cells in the rat brain prior to neurodegeneration. Acta Neuropathol Commun. 2017;5(1):85. doi: 10.1186/s40478-017-0494-9
- Chmielarz P, Domanskyi A. Alpha-synuclein preformed fibrils: A tool to understand Parkinson’s disease and develop disease modifying therapy. Neural Regen Res. 2021;16(11):2219-2221. doi: 10.4103/1673-5374.310686
- Polinski NK, Volpicelli-Daley LA, Sortwell CE, et al. Best practices for generating and using alpha-synuclein pre-formed fibrils to model Parkinson’s disease in rodents. J Parkinsons Dis. 2018;8(2):303-322. doi: 10.3233/JPD-171248
- Simola N, Morelli M, Carta AR. The 6-hydroxydopamine model of Parkinson’s disease. Neurotox Res. 2007;11(3- 4):151-167. doi: 10.1007/BF03033565
- Ferreira AFF, Binda KH, Real CC. The effects of treadmill exercise in animal models of Parkinson’s disease: A systematic review. Neurosci Biobehav Rev. 2021;131:1056-1075. doi: 10.1016/j.neubiorev.2021.10.019
- Glinka Y, Gassen M, Youdim MB. Mechanism of 6-hydroxydopamine neurotoxicity. J Neural Transm Suppl. 1997;50:55-66. doi: 10.1007/978-3-7091-6842-4_7
- Kupsch A, Schmidt W, Gizatullina Z, et al. 6-Hydroxydopamine impairs mitochondrial function in the rat model of Parkinson’s disease: Respirometric, histological, and behavioral analyses. J Neural Transm. 2014;121(10):1245-1257. doi: 10.1007/s00702-014-1185-3
- Berry C, La Vecchia C, Nicotera P. Paraquat and Parkinson’s disease. Cell Death Differ. 2010;17(7):1115-1125. doi: 10.1038/cdd.2009.217
- Manning-Bog AB, McCormack AL, Li J, Uversky VN, Fink AL, Di Monte DA. The herbicide paraquat causes up-regulation and aggregation of alpha-synuclein in mice: Paraquat and alpha-synuclein. J Biol Chem. 2002;277(3):1641-1644. doi: 10.1074/jbc.C100560200
- McCormack AL, Thiruchelvam M, Manning-Bog AB, et al. Environmental Risk factors and Parkinson’s disease: Selective degeneration of nigral dopaminergic neurons caused by the herbicide paraquat. Neurobiol Dis. 2002;10(2):119-127. doi: 10.1006/nbdi.2002.0507
- Betarbet R, Sherer TB, MacKenzie G, Garcia-Osuna M, Panov AV, Greenamyre JT. Chronic systemic pesticide exposure reproduces features of Parkinson’s disease. Nat Neurosci. 2000;3(12):1301-1306. doi: 10.1038/81834
- Innos J, Hickey MA. Using rotenone to model Parkinson’s disease in mice: A review of the role of pharmacokinetics. Chem Res Toxicol. 2021;34(5):1223-1239. doi: 10.1021/acs.chemrestox.0c00522
- Niederberger E, Wilken-Schmitz A, Manderscheid C, Schreiber Y, Gurke R, Tegeder I. Non-reproducibility of oral rotenone as a model for Parkinson’s disease in mice. Int J Mol Sci. 2022;23(20):12658. doi: 10.3390/ijms232012658
- Richter F, Hamann M, Richter A. Chronic rotenone treatment induces behavioral effects but no pathological signs of parkinsonism in mice. J Neurosci Res. 2007;85(3):681-691. doi: 10.1002/jnr.21159
- Inden M, Kitamura Y, Takeuchi H, et al. Neurodegeneration of mouse nigrostriatal dopaminergic system induced by repeated oral administration of rotenone is prevented by 4‐phenylbutyrate, a chemical chaperone. J Neurochem. 2007;101(6):1491-1504. doi: 10.1111/j.1471-4159.2006.04440.x
- Miyazaki I, Isooka N, Wada K, Kikuoka R, Kitamura Y, Asanuma M. Effects of enteric environmental modification by coffee components on neurodegeneration in rotenone-treated mice. Cells. 2019;8(3):221. doi: 10.3390/cells8030221
- McQueen EG, Doyle AE, Smirk FH. Mechanism of hypotensive action of reserpine, an alkaloid of Rauwolfia serpentina. Nature. 1954;174(4439):1015. doi: 10.1038/1741015b0
- Leão AHFF, Sarmento-Silva AJ, Santos JR, Ribeiro AM, Silva RH. Molecular, neurochemical, and behavioral hallmarks of reserpine as a model for Parkinson’s disease: New perspectives to a long-standing model. Brain Pathol. 2015;25(4):377-390. doi: 10.1111/bpa.12253
- Rijntjes M, Meyer PT. No free lunch with herbal preparations: Lessons from a case of parkinsonism and depression due to herbal medicine containing reserpine. Front Neurol. 2019;10:634. doi: 10.3389/fneur.2019.00634
- Dey P, Kundu A, Kumar A, et al. Analysis of alkaloids (indole alkaloids, isoquinoline alkaloids, tropane alkaloids). In: Recent Advances in Natural Products Analysis. Netherlands: Elsevier; 2020. p. 505-567. doi: 10.1016/B978-0-12-816455-6.00015-9
- Freis ED. Mental depression in hypertensive patients treated for long periods with large doses of reserpine. N Engl J Med. 1954;251(25):1006-1008. doi: 10.1056/NEJM195412162512504
- Steg G. Alpha-rigidity in reserpinized rats. Experientia. 1964;20(2):79-80. doi: 10.1007/BF02151251
- Carlsson M, Carlsson A. Marked locomotor stimulation in monoamine-depleted mice following treatment with atropine in combination with clonidine. J Neural Transm Park Dis Dement Sect. 1989;1(4):317-322. doi: 10.1007/BF02263486
- Carlsson A, Lindqvist M, Magnusson T. 3,4-Dihydroxyphenylalanine and 5-hydroxytryptophan as reserpine antagonists. Nature. 1957;180(4596):1200. doi: 10.1038/1801200a0
- Duty S, Jenner P. Animal models of Parkinson’s disease: A source of novel treatments and clues to the cause of the disease. Br J Pharmacol. 2011;164(4):1357-1391. doi: 10.1111/j.1476-5381.2011.01426.x
- Takashiba S, Van Dyke TE, Amar S, Murayama Y, Soskolne AW, Shapira L. Differentiation of monocytes to macrophages primes cells for lipopolysaccharide stimulation via accumulation of cytoplasmic nuclear factor kappaB. Infect Immun. 1999;67(11):5573-5578. doi: 10.1128/IAI.67.11.5573-5578.1999
- Wang Q, Liu Y, Zhou J. Neuroinflammation in Parkinson’s disease and its potential as therapeutic target. Transl Neurodegener. 2015;4:19. doi: 10.1186/s40035-015-0042-0
- Herrera AJ, Castaño A, Venero JL, Cano J, Machado A. The single intranigral injection of LPS as a new model for studying the selective effects of inflammatory reactions on dopaminergic system. Neurobiol Dis. 2000;7(4):429-447. doi: 10.1006/nbdi.2000.0289
- Iravani MM, Leung CCM, Sadeghian M, Haddon CO, Rose S, Jenner P. The acute and the long-term effects of nigral lipopolysaccharide administration on dopaminergic dysfunction and glial cell activation. Eur J Neurosci. 2005;22(2):317-330. doi: 10.1111/j.1460-9568.2005.04220.x
- Qin L, Wu X, Block ML, et al. Systemic LPS causes chronic neuroinflammation and progressive neurodegeneration. Glia. 2007;55(5):453-462. doi: 10.1002/glia.20467
- Waku I, Magalhães MS, Alves CO, de Oliveira AR. Haloperidol-induced catalepsy as an animal model for parkinsonism: A systematic review of experimental studies. Eur J Neurosci. 2021;53(11):3743-3767. doi: 10.1111/ejn.15222
- Luthra PM, Barodia SK, Raghubir R. Antagonism of haloperidol-induced swim impairment in l-dopa and caffeine treated mice: A pre-clinical model to study Parkinson’s disease. J Neurosci Methods. 2009;178(2):284-290. doi: 10.1016/j.jneumeth.2008.12.019
- Atack JR, Shook BC, Rassnick S, et al. JNJ-40255293, a novel adenosine A 2A/A 1 antagonist with efficacy in preclinical models of Parkinson’s disease. ACS Chem Neurosci. 2014;5(10):1005-1019. doi: 10.1021/cn5001606
- Bollinger SR, Engers DW, Panarese JD, et al. Discovery, structure-activity relationship, and biological characterization of a novel series of 6-((1 H -Pyrazolo[4,3- b ] pyridin-3-yl)amino)-benzo[ d ]isothiazole-3-carboxamides as positive allosteric modulators of the metabotropic glutamate receptor 4. J Med Chem. 2019;62(1):342-358. doi: 10.1021/acs.jmedchem.8b00994
- Gasser T, Hardy J, Mizuno Y. Milestones in PD genetics. Mov Disord. 2011;26(6):1042-1048. doi: 10.1002/mds.23637
- Dehay B, Bourdenx M, Gorry P, et al. Targeting α-synuclein for treatment of Parkinson’s disease: Mechanistic and therapeutic considerations. Lancet Neurol. 2015;14(8):855-866. doi: 10.1016/S1474-4422(15)00006-X
- Chesselet MF, Fleming S, Mortazavi F, Meurers B. Strengths and limitations of genetic mouse models of Parkinson’s disease. Parkinsonism Relat Disord. 2008;14 Suppl 2(Suppl 2):S84-S87. doi: 10.1016/j.parkreldis.2008.04.004
- Blesa J, Przedborski S. Parkinson’s disease: Animal models and dopaminergic cell vulnerability. Front Neuroanat. 2014;8:155. doi: 10.3389/fnana.2014.00155
- Lin X, Parisiadou L, Sgobio C, et al. Conditional expression of Parkinson’s disease-related mutant α-synuclein in the midbrain dopaminergic neurons causes progressive neurodegeneration and degradation of transcription factor nuclear receptor related 1. J Neurosci. 2012;32(27):9248-9264. doi: 10.1523/JNEUROSCI.1731-12.2012
- Kitada T, Asakawa S, Hattori N, et al. Mutations in the parkin gene cause autosomal recessive juvenile parkinsonism. Nature. 1998;392(6676):605-608. doi: 10.1038/33416
- Zhang Y, Gao J, Chung KK, Huang H, Dawson VL, Dawson TM. Parkin functions as an E2-dependent ubiquitin- protein ligase and promotes the degradation of the synaptic vesicle-associated protein, CDCrel-1. Proc Natl Acad Sci U S A. 2000;97(24):13354-13359. doi: 10.1073/pnas.240347797
- Dawson TM, Dawson VL. The role of parkin in familial and sporadic Parkinson’s disease. Mov Disord. 2010;25 Suppl 1(1):S32-S39. doi: 10.1002/mds.22798
- Kin K, Yasuhara T, Kameda M, Date I. Animal models for Parkinson’s disease research: Trends in the 2000s. Int J Mol Sci. 2019;20(21):5402. doi: 10.3390/ijms20215402
- Narendra DP, Jin SM, Tanaka A, et al. PINK1 is selectively stabilized on impaired mitochondria to activate Parkin. PLoS Biol. 2010;8(1):e1000298. doi: 10.1371/journal.pbio.1000298
- Schneider SA, Alcalay RN. Neuropathology of genetic synucleinopathies with parkinsonism: Review of the literature. Mov Disord. 2017;32(11):1504-1523. doi: 10.1002/mds.27193
- Dawson TM, Ko HS, Dawson VL. Genetic animal models of Parkinson’s disease. Neuron. 2010;66(5):646-661. doi: 10.1016/j.neuron.2010.04.034
- Perez FA, Palmiter RD. Parkin-deficient mice are not a robust model of parkinsonism. Proc Natl Acad Sci. 2005;102(6):2174-2179. doi: 10.1073/pnas.0409598102
- Paul S, Pickrell AM. Hidden phenotypes of PINK1/ Parkin knockout mice. Biochim Biophys Acta Gen Subj. 2021;1865(6):129871. doi: 10.1016/j.bbagen.2021.129871
- Kitada T, Tong Y, Gautier CA, Shen J. Absence of nigral degeneration in aged parkin/DJ‐1/PINK1 triple knockout mice. J Neurochem. 2009;111(3):696-702. doi: 10.1111/j.1471-4159.2009.06350.x
- Noda S, Sato S, Fukuda T, et al. Loss of Parkin contributes to mitochondrial turnover and dopaminergic neuronal loss in aged mice. Neurobiol Dis. 2020;136:104717. doi: 10.1016/j.nbd.2019.104717
- Wallings R, Manzoni C, Bandopadhyay R. Cellular processes associated with LRRK2 function and dysfunction. FEBS J. 2015;282(15):2806-2826. doi: 10.1111/febs.13305
- Zimprich A, Biskup S, Leitner P, et al. Mutations in LRRK2 cause autosomal-dominant parkinsonism with pleomorphic pathology. Neuron. 2004;44(4):601-607. doi: 10.1016/j.neuron.2004.11.005
- Volta M, Melrose H. LRRK2 mouse models: Dissecting the behavior, striatal neurochemistry and neurophysiology of PD pathogenesis. Biochem Soc Trans. 2017;45(1):113-122. doi: 10.1042/BST20160238
- Lee Y, Dawson VL, Dawson TM. Animal models of Parkinson’s disease: Vertebrate genetics. Cold Spring Harb Perspect Med. 2012;2(10):a009324. doi: 10.1101/cshperspect.a009324
- Dovonou A, Bolduc C, Soto Linan V, Gora C, Peralta Iii MR, Lévesque M. Animal models of Parkinson’s disease: Bridging the gap between disease hallmarks and research questions. Transl Neurodegener. 2023;12(1):36. doi: 10.1186/s40035-023-00368-8
- Ramonet D, Daher JPL, Lin BM, et al. Dopaminergic neuronal loss, reduced neurite complexity and autophagic abnormalities in transgenic mice expressing G2019S mutant LRRK2. PLoS One. 2011;6(4):e18568. doi: 10.1371/journal.pone.0018568
- Chasseigneaux S, Allinquant B. Functions of Aβ, sAPPα and sAPPβ : Similarities and differences. J Neurochem. 2012;120(s1):99-108. doi: 10.1111/j.1471-4159.2011.07584.x
- De Strooper B, Iwatsubo T, Wolfe MS. Presenilins and γ-secretase: Structure, function, and role in Alzheimer disease. Cold Spring Harb Perspect Med. 2012;2(1):a006304. doi: 10.1101/cshperspect.a006304
- Radde R, Bolmont T, Kaeser SA, et al. Aβ42‐driven cerebral amyloidosis in transgenic mice reveals early and robust pathology. EMBO Rep. 2006;7(9):940-946. doi: 10.1038/sj.embor.7400784
- Richard BC, Kurdakova A, Baches S, Bayer TA, Weggen S, Wirths O. Gene dosage dependent aggravation of the neurological phenotype in the 5×FAD mouse model of Alzheimer’s disease. J Alzheimers Dis. 2015;45(4):1223-1236. doi: 10.3233/JAD-143120
- Giannoni P, Arango-Lievano M, Neves ID, et al. Cerebrovascular pathology during the progression of experimental Alzheimer’s disease. Neurobiol Dis. 2016;88:107-117. doi: 10.1016/j.nbd.2016.01.001
- Oblak AL, Lin PB, Kotredes KP, et al. Comprehensive evaluation of the 5×FAD mouse model for preclinical testing applications: A MODEL-AD study. Front Aging Neurosci. 2021;13:713726. doi: 10.3389/fnagi.2021.713726
- Jawhar S, Trawicka A, Jenneckens C, Bayer TA, Wirths O. Motor deficits, neuron loss, and reduced anxiety coinciding with axonal degeneration and intraneuronal Aβ aggregation in the 5×FAD mouse model of Alzheimer’s disease. Neurobiol Aging. 2012;33(1):196.e29-e40. doi: 10.1016/j.neurobiolaging.2010.05.027
- Mucke L, Masliah E, Yu GQ, et al. High-level neuronal expression of abeta 1-42 in wild-type human amyloid protein precursor transgenic mice: Synaptotoxicity without plaque formation. J Neurosci. 2000;20(11):4050-4058. doi: 10.1523/JNEUROSCI.20-11-04050.2000
- Wright AL, Zinn R, Hohensinn B, et al. Neuroinflammation and neuronal loss precede Aβ plaque deposition in the hAPP-J20 mouse model of Alzheimer’s disease. PLoS One. 2013;8(4):e59586. doi: 10.1371/journal.pone.0059586
- Jankowsky JL, Slunt HH, Gonzales V, et al. Persistent amyloidosis following suppression of Abeta production in a transgenic model of Alzheimer disease. PLoS Med. 2005;2(12):e355. doi: 10.1371/journal.pmed.0020355
- Ben-Nejma IRH, Keliris AJ, Vanreusel V, Ponsaerts P, Van der Linden A, Keliris GA. Altered dynamics of glymphatic flow in a mature-onset Tet-off APP mouse model of amyloidosis. Alzheimers Res Ther. 2023;15(1):23. doi: 10.1186/s13195-023-01175-z
- Ben-Nejma IRH, Keliris AJ, Daans J, et al. Increased soluble amyloid-beta causes early aberrant brain network hypersynchronisation in a mature-onset mouse model of amyloidosis. Acta Neuropathol Commun. 2019;7(1):180. doi: 10.1186/s40478-019-0810-7
- Tomiyama T, Matsuyama S, Iso H, et al. A mouse model of amyloid beta oligomers: Their contribution to synaptic alteration, abnormal tau phosphorylation, glial activation, and neuronal loss in vivo. J Neurosci. 2010;30(14):4845-4856. doi: 10.1523/JNEUROSCI.5825-09.2010
- Oddo S, Caccamo A, Shepherd JD, et al. Triple-transgenic model of Alzheimer’s disease with plaques and tangles: Intracellular Abeta and synaptic dysfunction. Neuron. 2003;39(3):409-421. doi: 10.1016/s0896-6273(03)00434-3
- Guo Q, Fu W, Sopher BL, et al. Increased vulnerability of hippocampal neurons to excitotoxic necrosis in presenilin-1 mutant knock-in mice. Nat Med. 1999;5(1):101-106. doi: 10.1038/4789
- Stover KR, Campbell MA, Van Winssen CM, Brown RE. Early detection of cognitive deficits in the 3×Tg-AD mouse model of Alzheimer’s disease. Behav Brain Res. 2015;289:29-38. doi: 10.1016/j.bbr.2015.04.012
- Saito T, Matsuba Y, Mihira N, et al. Single App knock-in mouse models of Alzheimer’s disease. Nat Neurosci. 2014;17(5):661-663. doi: 10.1038/nn.3697
- Nilsson P, Saito T, Saido TC. New mouse model of Alzheimer’s. ACS Chem Neurosci. 2014;5(7):499-502. doi: 10.1021/cn500105p
- Latif-Hernandez A, Sabanov V, Ahmed T, et al. The two faces of synaptic failure in AppNL-G-F knock-in mice. Alzheimers Res Ther. 2020;12(1):100. doi: 10.1186/s13195-020-00667-6
- Foley KE, Hewes AA, Garceau DT, et al. The APOE ε3/ε4 genotype drives distinct Gene signatures in the cortex of young mice. Front Aging Neurosci. 2022;14:838436. doi: 10.3389/fnagi.2022.838436
- McLean JW, Bhattrai A, Vitali F, Raikes AC, Wiegand JPL, Brinton RD. Contributions of sex and genotype to exploratory behavior differences in an aged humanized APOE mouse model of late-onset Alzheimer’s disease. Learn Mem. 2022;29(9):321-331. doi: 10.1101/lm.053588.122
- Huynh TP V, Wang C, Tran AC, et al. Lack of hepatic apoE does not influence early Aβ deposition: Observations from a new APOE knock-in model. Mol Neurodegener. 2019;14(1):37. doi: 10.1186/s13024-019-0337-1
- Piedrahita JA, Zhang SH, Hagaman JR, Oliver PM, Maeda N. Generation of mice carrying a mutant apolipoprotein E gene inactivated by gene targeting in embryonic stem cells. Proc Natl Acad Sci. 1992;89(10):4471-4475. doi: 10.1073/pnas.89.10.4471
- Denk F, Wade-Martins R. Knock-out and transgenic mouse models of tauopathies. Neurobiol Aging. 2009;30(1):1-13. doi: 10.1016/j.neurobiolaging.2007.05.010
- Andorfer C, Kress Y, Espinoza M, et al. Hyperphosphorylation and aggregation of tau in mice expressing normal human tau isoforms. J Neurochem. 2003;86(3):582-590. doi: 10.1046/j.1471-4159.2003.01879.x
- Morley JE. The SAMP8 mouse: A model of Alzheimer disease? Biogerontology. 2002;3(1-2):57-60. doi: 10.1023/a:1015207429786
- Miyamoto M, Kiyota Y, Yamazaki N, et al. Age-related changes in learning and memory in the senescence-accelerated mouse (SAM). Physiol Behav. 1986;38(3):399-406. doi: 10.1016/0031-9384(86)90112-5
- Yagi H, Katoh S, Akiguchi I, Takeda T. Age-related deterioration of ability of acquisition in memory and learning in senescence accelerated mouse: SAM-P/8 as an animal model of disturbances in recent memory. Brain Res. 1988;474(1):86-93. doi: 10.1016/0006-8993(88)90671-3
- Liu B, Liu J, Shi JS. SAMP8 mice as a model of age-related cognition decline with underlying mechanisms in Alzheimer’s disease. J Alzheimers Dis. 2020;75(2):385-395. doi: 10.3233/JAD-200063
- Capdevila J, Ducreux M, García Carbonero R, et al. Streptozotocin, 1982-2022: forty years from the FDA’s approval to treat pancreatic neuroendocrine tumors. Neuroendocrinology. 2022;112(12):1155-1167. doi: 10.1159/000524988
- Mostafavinia A, Amini A, Ghorishi SK, Pouriran R, Bayat M. The effects of dosage and the routes of administrations of streptozotocin and alloxan on induction rate of type1 diabetes mellitus and mortality rate in rats. Lab Anim Res. 2016;32(3):160-165. doi: 10.5625/lar.2016.32.3.160
- Grieb P. Intracerebroventricular streptozotocin injections as a model of Alzheimer’s disease: In search of a relevant mechanism. Mol Neurobiol. 2016;53(3):1741-1752. doi: 10.1007/s12035-015-9132-3
- Akhtar A, Sah SP. Insulin signaling pathway and related molecules: Role in neurodegeneration and Alzheimer’s disease. Neurochem Int. 2020;135:104707. doi: 10.1016/j.neuint.2020.104707
- Akhtar A, Gupta SM, Dwivedi S, Kumar D, Shaikh MF, Negi A. Preclinical models for Alzheimer’s disease: Past, present, and future approaches. ACS Omega. 2022;7(51):47504-47517. doi: 10.1021/acsomega.2c05609
- Dhapola R, Kumari S, Sharma P, HariKrishnaReddy D. Insight into the emerging and common experimental in-vivo models of Alzheimer’s disease. Lab Anim Res. 2023;39(1):33. doi: 10.1186/s42826-023-00184-1
- Ravelli KG, Rosário BDA, Camarini R, Hernandes MS, Britto LR. Intracerebroventricular streptozotocin as a model of Alzheimer’s disease: Neurochemical and behavioral characterization in mice. Neurotox Res. 2017;31(3):327-333. doi: 10.1007/s12640-016-9684-7
- Rapaka D, Adiukwu PC, Bitra VR. Experimentally induced animal models for cognitive dysfunction and Alzheimer’s disease. MethodsX. 2022;9:101933. doi: 10.1016/j.mex.2022.101933
- Eisele YS, Bolmont T, Heikenwalder M, et al. Induction of cerebral β-amyloidosis: Intracerebral versus systemic Aβ inoculation. Proc Natl Acad Sci. 2009;106(31):12926-12931. doi: 10.1073/pnas.0903200106
- McLarnon JG. Correlated inflammatory responses and neurodegeneration in peptide-injected animal models of Alzheimer’s disease. Biomed Res Int. 2014;2014:923670. doi: 10.1155/2014/923670
- Zhang HY, Yan H, Tang XC. Huperzine A enhances the level of secretory amyloid precursor protein and protein kinase C-alpha in intracerebroventricular beta-amyloid-(1-40) infused rats and human embryonic kidney 293 Swedish mutant cells. Neurosci Lett. 2004;360(1-2):21-24. doi: 10.1016/j.neulet.2004.01.055
- Shekarian M, Komaki A, Shahidi S, Sarihi A, Salehi I, Raoufi S. The protective and therapeutic effects of vinpocetine, a PDE1 inhibitor, on oxidative stress and learning and memory impairment induced by an intracerebroventricular (ICV) injection of amyloid beta (aβ) peptide. Behav Brain Res. 2020;383:112512. doi: 10.1016/j.bbr.2020.112512
- Yin S, Ran Q, Yang J, Zhao Y, Li C. Nootropic effect of neferine on aluminium chloride-induced Alzheimer’s disease in experimental models. J Biochem Mol Toxicol. 2020;34(2):e22429. doi: 10.1002/jbt.22429
- Hethey C, Hartung N, Wangorsch G, Weisser K, Huisinga W. Physiology-based toxicokinetic modelling of aluminium in rat and man. Arch Toxicol. 2021;95(9):2977-3000. doi: 10.1007/s00204-021-03107-y
- Praticò D, Uryu K, Sung S, Tang S, Trojanowski JQ, Lee VMY. Aluminum modulates brain amyloidosis through oxidative stress in APP transgenic mice. FASEB J. 2002;16(9):1138-1140. doi: 10.1096/fj.02-0012fje
- Wohleb ES, Gerhard D, Thomas A, Duman RS. Molecular and cellular mechanisms of rapid-acting antidepressants ketamine and scopolamine. Curr Neuropharmacol. 2017;15(1):11-20. doi: 10.2174/1570159X14666160309114549
- Bajo R, Pusil S, López ME, et al. Scopolamine effects on functional brain connectivity: A pharmacological model of Alzheimer’s disease. Sci Rep. 2015;5(1):9748. doi: 10.1038/srep09748
- Chen WN, Yeong KY. Scopolamine, a toxin-induced experimental model, used for research in Alzheimer’s disease. CNS Neurol Disord Drug Targets. 2020;19(2):85-93. doi: 10.2174/1871527319666200214104331